5 Fundamentals of Tissue Engineering
Introduction
Craniofacial reconstruction represents an important sector of facial plastic surgery and accounts for a significant portion of cases performed annually. The American Society of Plastic Surgeons recently reported an annual approximation of 195,400 maxillofacial procedures, 243,800 rhinoplasty and nasal reconstructive cases, and 26,450 ear reconstructive procedures. The American Academy of Facial Plastic and Reconstructive Surgery reported approximately 143,100 rhinoplasty and nasal reconstructive procedures within the same year. In nasal reconstruction, specifically, surgery is performed to meet either of two primary objectives: restoration of nasal function and refinement of the aesthetic appearance of the nose. Both objectives frequently target shared anatomical deficiencies or deformities that must be corrected. This may mean rebuilding weak, malpositioned, or lost nasal framework components. The cause may be congenital but frequently is acquired from trauma, malignancy, or an iatrogenic alteration such as prior rhinoplasty. Unlike bone, cartilage possesses little innate ability for repair and regeneration. Alterations in the nasal skeletal framework can, therefore, lead to permanent loss of structure and function and pose a considerable reconstructive challenge.
In the properly functioning nose, gravitational and elastic forces are present within the nasal skin, muscle, and the fibrocartilaginous connections in between the cartilage components. The flexural properties of the hyaline cartilage of the nasal framework provide springlike resistance to transient external loading, and the nasal pyramid distributes the internal and external compressive forces. Restoration of the aesthetic and functional properties of the nose, therefore, relies upon recreating the flexural support structure provided by the shaped cartilaginous framework of the nose. To effectively support the nasal framework, the properties of a structural grafting material must include adequate shape fidelity and appropriate loadbearing potential. Historically, rhinoplasty procedures favored subtractive approaches to nasal reconstruction; however, it has become evident that a tissue preserving, restorative approach to the nasal framework is preferable. Numerous grafting options are available, ranging from a variety of alloplastic implants, allografts, and autologous tissue sources. Although each may be employed with success, autologous cartilage grafts are ideal for most reconstructive goals and remain the most commonly used tissue source today. 1
Cartilage grafting for nasal reconstruction became popular in the 1940s when Peer 2 reported the finding that autologous septal and auricular cartilage grafts are resistant to resorption. Compared to bone grafts, autologous cartilage grafts have lower metabolic requirements for survival. 3 Septal, auricular, and rib cartilage grafts may all be utilized in nasal reconstruction; however, septal cartilage possesses distinct advantages over auricular and rib cartilage. The tissue has considerable ease of harvest, minimal donor site morbidity, and has superior structural properties that help to defy the contractile forces of the skin–soft tissue envelope during the healing period. Unlike auricular cartilage, septal cartilage is firm yet flexible, allowing the surgeon to carve and shape the cartilage to suit the specific reconstructive needs of the patient. Auricular cartilage, on the other hand, is structurally weaker than either septal or costal cartilage although its ease of harvest makes it a reasonable choice when adequate septal cartilage is not available. 4 Rib cartilage, which provides considerable tissue volume and has greater tensile strength than septal cartilage, is associated with greater donor site morbidity and also can warp after implantation due to the structural weakening caused by carving of the implant. 3
Alternatives to autologous cartilage include a wide variety of allogenic grafts such as irradiated rib, cadaveric dermis, and hydroxyapatite cement as well as alloplastic grafts including silicone, high-density polyethylene, and polytetrafluoroethylene (PTFE). Although these remain widely available and offer no donor site morbidity, there are notable risks and important shortcomings associated with their use. 5 , 6 , 7 , 8 Allogenic grafts carry the risk of resorption, immune rejection, and disease transmission. Synthetic grafts may be complicated by infection and extrusion. For these reasons autologous cartilage remains the preferred grafting material in the majority of situations. The volume of tissue available for harvest, however, becomes an important consideration. Prior aesthetic or reconstructive surgery involving septal or auricular cartilage harvest limits the tissue volume available for subsequent harvest and corrective surgery. This redirects the surgeon to harvesting autologous rib or using an allogenic graft material. Rather than resorting to these other tissue sources, cartilage tissue engineering offers the potential to generate large quantities of autologous cartilage from a small donor specimen and create grafts in shapes and sizes that match the reconstructive needs of the patient. This chapter details the process and state of the art of cartilage tissue engineering as well as the future challenges and opportunities within the field.
Structural Components and Physical Properties of Cartilage
The utility of various cartilage sources as grafts depends upon the type of cartilage and, therefore, the structure and biochemical makeup. Nasal septal and rib cartilage share a similar composition as both are hyaline cartilage (as are articular, tracheal, and bronchial cartilage) ( Fig. 5.1 ). Elastic cartilage is found in the auricle, the epiglottis, and the fibrocartilage of the temporomandibular joint, menisci of the knee, and the nucleus pulposus of intervertebral disks. Hyaline cartilage contains primarily type II collagen as does elastic cartilage which has a network of elastin to provide greater flexibility and less rigidity. Fibrocartilage, on the other hand, is primarily made up of bundles of large type I collagen fibers that allow for excellent shock absorption.

Investigation of the biochemical composition of hyaline cartilage demonstrates that absolute and relative abundance of certain structural molecules in the extracellular matrix confers the biomechanical properties of the tissue. 9 The tensile modulus and strength of hyaline cartilage are attributed primarily to the collagen network 9 , 10 , 11 whereas it is the glycosaminoglycan (GAG) content that allows the cartilage to resist compression. In the native adult human nasal septum, the collagen content is approximately 4.4-fold that of GAG content. 12 , 13 , 14 Maintaining an adequate ratio of collagen to GAG in tissueengineered cartilage is paramount in producing neocartilage constructs with adequate mechanical strength and stiffness for surgical implantation.
The mechanical characteristics of native septal cartilage are well described and have been measured in terms of tensile, compressive, and flexural properties each of which confer unique attributes to the behavior of the tissue as an implant. 13 , 14 , 15 Tensile testing provides a measure of strength and stiffness by recording its resistance to stretch. Compression testing, on the other hand, provides a measure of loadbearing ability. Flexural testing assesses tissue rigidity which is important given the natural curvature of facial contours and day to day bending and deformation of facial structures.
Tissue engineering efforts have sought to match the native biochemical and biomechanical properties of native septal tissue. Progress continues, yet this has proven to be a formidable task. Unlike implants used for orthopedic purposes, cartilage implants in facial reconstruction require far more limited loadbearing capacity and are not subjected to extreme physiologic forces. Zemek et al sought to develop target specifications for human septal neocartilage prototypes by producing urethane phantoms mimicking actual cartilage grafts in shape and size but with variable elastic properties. 16 Minimal acceptable stiffness for columellar, L-strut, and alar graft applications was determined by a group of blinded surgeons who handled and evaluated the phantoms. Manufacturing challenges as well as cost and time increase with the need for greater stiffness; therefore, it becomes critical to establish the minimal mechanical stability of implants for nasal reconstruction. These findings have established concrete design goals for bioengineered constructs. Table 5.1 summarizes the known properties of native septal cartilage, the properties of septal neocartilage constructs (produced after 4 weeks of culture in a bioreactor), and the design goals of prototypical engineered septal constructs that would have the desirable composition, mechanical properties, and applicable geometry for surgical implantation. Of particular note is the collagen to GAG ratio, which is critical to biomechanical performance, and in tissueengineered implants has considerably lower values than both native septal cartilage and the neocartilage prototype.
Tissue Engineering of Cartilage
Choosing a Chondrocytes Source
Cartilage was one of the first tissues to be investigated in early tissue engineering efforts. 17 It possesses specific traits that make manufacturing and implantation of tissueengineered cartilage far more feasible than other bodily tissues. Firstly, it consists of a single cell type unlike skin and most other tissue types. Secondly, cartilage is a thin, avascular tissue that exchanges nutrients, waste, and gasses by diffusion. This simplifies tissue culture and it also means that implantation is more straightforward since incorporation into host tissues does not require neovascularization for success. 17
Potential donor sites for cartilage tissue engineering in facial reconstruction include the nasal septum, auricle, and rib. Articular cartilage is considered far less practical for tissue donation as the morbidity of harvest is considerably greater. Investigation of septal, auricular, and rib chondrocytes for tissue engineering have consistently demonstrated the superiority of septal chondrocytes for several reasons. Tay et al examined the comparative suitability of nasal septal, auricular, and costal cartilage in generating autologous tissueengineered cartilage in threedimensional pellets. Nasal septal and auricular cartilage grafts demonstrated greater redifferentiation to a productive chondrocyte phenotype than costal grafts and, therefore, better quality cartilage as measured by production of GAG and type II collagen. 18
Neumann et al compared cartilage from nasal septal and auricular tissue sources that were expanded in monolayer culture then seeded into a cell culture insert containing a semipermeable membrane. The septum-derived engineered cartilage was preferable in that it demonstrated greater total GAG content within the extracellular matrix (ECM). 19 Tissue engineering with septal chondrocytes has also been compared to utilization of articular chondrocytes, which is well described from the vast body of research in articular cartilage tissue engineering. Kafienah et al showed that human nasal septal chondrocytes proliferated four times faster and had significantly higher chondrogenic capacity than articular chondrocytes. 20 Taken together, these studies suggest that human nasal septal chondrocytes offer the greatest potential for engineering of cartilage constructs to be used in facial reconstructive surgery.
Chondrocyte Expansion in Monolayer Culture
The process of tissue engineering begins with harvesting cartilage from the donor patient. The cartilage is then enzymatically digested to isolate the chondrocytes. Only a limited number of chondrocytes are required to be harvested because the chondrocyte population will undergo exponential expansion when spread over a flat surface in media as a monolayer. During monolayer expansion, the chondrocytes undergo a process of dedifferentiation in which their phenotypic appearance and synthetic output shifts toward that of a fibroblast. 21 , 22 The typical round, polygonal appearance of the chondrocytes becomes flattened and spindle-shaped and synthetic output shifts from type II to primarily type I collagen ( Fig. 5.2 ). Once the cell population has been adequately expanded, it is transferred from the twodimensional flat surface to a threedimensional (3D) gel or scaffold system to promote redifferentiation into the chondrocytic phenotype. The cells return to a polygonal cell shape, shift their synthetic output from type I to type II collagen production (typical of hyaline cartilage), and proliferate at a lower rate. 4 , 12 , 23 This phenotype is maintained as the cells lay down ECM that will ultimately be part of the final tissueengineered cartilage construct.

Threedimensional Culture Systems
After a chondrocyte population has been adequately expanded, the cells are transferred to one of a variety of different 3D culture systems that will support chondrogenesis and formation of a tissue construct. Early work in the field of cartilage tissue engineering focused on culture systems that utilize biodegradable scaffolds to suspend cells in a 3D matrix. 24 Scaffolds have been created out of various materials, the most popular of which have been poly-L-lactic acid, polyglycolic acid, and numerous copolymers. 25 , 26 , 27 The scaffolds provide a woven mesh that traps free chondrocytes, allows cell adherence, and promotes cell–cell interactions within a 3D configuration. Conceptually biodegradable scaffolds provide an ideal means to generate tissue of various shapes, sizes, and thicknesses. However, in practice, this technology has met several limitations. One reason is that an effective structural framework must possess a number of critical properties, many of which have been difficult to achieve. For example, a scaffold must be free of cytotoxins involved with fabrication, must not produce pro-inflammatory degradation byproducts, must degrade at a rate that does not outpace cartilaginous ingrowth, and must promote the differentiated chondrocytic phenotype and associated matrix synthesis. 28 Among these, sustaining the chondrocytic prosynthetic phenotype remains the greatest challenge and has only been achieved with limited success. Prior investigation has demonstrated that human nasal septal chondrocytes seeded into biodegradable scaffolds demonstrate poor construct structural integrity and promote fibrous rather than cartilaginous ECM deposition. 28 , 29 , 30 This is consistent with loss of the chondrogenic phenotype. It has been further noted that tissue scaffolds, despite being 3D, do not guarantee a similar tissue construct conformation. Histologic analysis of polyglycolic acid scaffolds seeded with human septal chondrocytes demonstrated that within the 3D scaffold the cellular expansion itself was twodimensional. 22
Current investigation in cartilage tissue engineering has, therefore, shifted toward scaffoldfree culture and seeks to achieve biochemical and biomechanical properties similar to native cartilage in the absence of a prefabricated framework for cellular organization. This is not dissimilar to the process that occurs in embryonic development in which signaling molecules and cell–cell interaction direct self-organization of cells into organized cartilaginous tissue. 28
Hydrogels, such as agarose and alginate, have been utilized successfully in allowing suspension of cells in 3D culture that promotes the chondrocytic phenotype. In just 4 weeks, agarose wells seeded with bovine articular chondrocytes demonstrated synthesis of neocartilage constructs that possessed morphological, biochemical, histological, and biomechanical similarity to native articular cartilage. 31 Alginate has gained particular favor, as it can be seeded with cells and then transformed from a liquid to a gel immediately in the presence of calcium through polymerization of its L-glucuronic acid and D-mannuronic acid constituents. This allows formation of small beads with chondrocytes suspended in culture within the gel ( Fig. 5.3 ). The alginate can then be depolymerized after the desired period of culture through the addition of a calcium chelator such as sodium citrate, which releases the redifferentiated chondrocytes and their pericellular matrix from the gel. The chondrocytes and associate matrix are then able to be transferred into semipermeable wells where they gradually mature into scaffoldfree disks of neocartilage.

This process was refined by Masuda et al in what has been called the alginate-recoveredchondrocyte (ARC) method. 32 , 33 , 34 First achieved with bovine articular chondrocytes, the method was subsequently repeated by Chia et al with success using human nasal septal chondrocytes. 35 The chondrocytes were first expanded in monolayer culture and then either seeded directly onto semipermeable inserts or cultured through the ARC method as an intermediate step prior to seeding on semipermeable inserts. The ARC constructs demonstrated the histologic and gross appearance of cartilage whereas the constructs that did not undergo ARC culture failed to achieve this phenotype and had significantly less collagen and GAG deposition.
Achieving the Prototype Goal
Considerable effort has focused on understanding the composition, structure, and mechanical properties of native human septal cartilage in order to establish benchmarks for the design of septal neocartilage construct prototypes. The minimal acceptable stiffness values for various implant applications have been further refined by Zemek et al with synthetic phantom implants as previously described. 16 Numerous strategies have been employed to improve construct quality to meet the biochemical and biomechanical specifications the ideal prototype. By precisely controlling the physical and chemical environment in tissue culture, the properties of tissueengineered cartilage can be optimized. A network of countless cytokines, hormones, and growth factors, each influential in chondrocytic signaling pathways, continues to be elucidated and remains critical in the design of the tissue culture environment.
Successful tissue engineering of cartilage constructs, as in all cell and tissue culture, requires nutritionally supplemented media to nourish the cells and support growth. The most widely used media supplement is fetal bovine serum (FBS) which has long been a mainstay of eukaryotic cell culture. 36 Its high levels of growth-stimulatory factors allow it to be used across a wide array of cell culture applications and it has been utilized in the great majority of human cartilage tissue engineering studies published to date.
More recent research, however, has demonstrated that supplementation with human serum (HS) may allow cells to reach greater proliferative and chondrogenic potential than has been achieved using FBS. 37 After 3D culture, comparison of the biomechanical properties of constructs grown in media containing HS versus FBS demonstrates those grown in HS to be superior. 35 Biochemically, constructs cultured in HS are consistently superior as well. Alexander et al reported that HS supplemented media yielded fourfold greater cellular proliferation and sixfold greater type II collagen/DNA ratio in nasal septal cartilage constructs than those grown in FBS supplemented media under otherwise identical conditions. 38
Considerable progress toward a prototype model has also been achieved through modulation of growth factors which have proven to be a key driving force in the chondrogenic pathway. In culture of human nasal septal chondrocytes, specifically, transforming growth factor beta 1(TGF-β1), fibroblast growth factor 1 and 2 (FGF-1,-2), insulin-like growth factor 1 (IGF-1), platelet-derived growth factor BB (PDGF-BB), and bone morphogenic protein-2 and -7 (BMP-2,-7) have all been shown to increase cellular proliferation during monolayer expansion. 18 , 20 , 39 , 40 , 41 , 42 Investigation of TGF-β and IGF-1 further demonstrate a stimulatory effect on synthesis of key ECM components GAG and type II collagen. 17 , 39 , 43 Growth factor combinations have also emerged as an important area of investigation as it becomes increasingly apparent that the process of chondrogenesis involves multiple overlapping chemical signals and regulatory pathways. Alexander et al demonstrated that media supplementation of nasal septal cartilage constructs with human serum, IGF-1, and growth differentiation factor 5 (GDF-5) produced constructs that were 12-fold thicker and had significantly more GAG and type II collagen accumulation than those developed in media with HS alone. The improved ECM production translated to improved biomechanical properties upon compression testing. 44
Both GAG and type II collagen deposition are critical but it is clear that it is the collagen to GAG ratio that correlates most strongly with mechanical strength and stiffness. 45 In addition to optimizing the biochemical milieu of culture media, it is possible to modulate this ratio through chemical extraction. A number of studies have demonstrated improved tensile properties of articular cartilage tissue after glycosidase-mediated GAG depletion. 46 , 47 , 48 , 49 Treatment of articular cartilage explants 49 and engineered articular cartilage 50 , 51 with the glycosidases chondroitinase-ABC (C-ABC) or Streptomyces hyaluronidase resulted in increased collagen content and improved tensile properties. 49 The mechanism of increase in tissue strength is unclear but one possible explanation is that proteoglycan depletion allows the collagen fibrils to associate linearly (endto-end) or laterally (side-toside) in a more organized manner, lending strength to the tissue. 52 , 53
It is clear that in addition to optimizing the biochemical culture environment, significant gains in the collagen to GAG ratios and mechanical tissue properties can be achieved by creating a dynamic physical environment during tissue maturation. Numerous types of dynamic culture systems, or bioreactors, have been developed with the premise of providing mechanical stimulation, mixing of media, and improved oxygen diffusion. They provide a homogeneous nutrient and oxygen concentration that minimizes tissue hypoxia and nutrient deprivation that may occur in static culture. Furthermore, it is becoming increasingly evident that mechanical and hydrodynamic forces transmitted in dynamic culture may exert a stimulatory influence on cell and tissue development. 17
Although various types of bioreactors remain in use, the best overall results have been achieved with rotatingwall bioreactors. 54 Initially conceptualized by NASA after encouraging tissue culture results in the microgravity of space, the culture vessels rotate on a horizontal axis to maintain constructs in continuous free-fall within the media ( Fig. 5.4 ). 55 Numerous studies have subsequently demonstrated the benefit of rotatingwall bioreactors in chondrogenesis. Sheehy et al demonstrated that dynamic rotational culture significantly increases accumulation of GAG and collagen in porcine chondrocytes as compared to constructs grown in static culture. 56 Furthermore, the deposition of GAG and collagen was more uniform throughout constructs cultured in rotational vessels, resulting in a more homogeneous and uniform tissue. Vunjak-Novakovic et al compared bovine articular chondrocytes cultured on polyglycolic acid scaffolds in static flasks, spinner bioreactors, and rotating bioreactors. 57 They found that constructs cultured in rotating bioreactors had the greatest GAG and collagen accumulation as well as superior mechanical properties. Similar results have been achieved with human articular chondrocytes seeded in alginate/chitosan microcapsules matured in a bioreactor environment. 58

Although utilized with success in articular cartilage tissue engineering, published data on the application of bioreactors in nasal septal tissue engineering has only recently begun to emerge. Gorti et al observed a more mechanically robust construct with handling when human septal chondrocytes were cultured in a rotating bioreactor for 6 weeks compared with constructs cultured in a spinner flask for 14 days. 59 More recently, Bichara et al demonstrated the benefit of bioreactor culture in a comparison of in vivo construct development with or without bioreactor culture prior to implantation. 60 After 6 weeks of in vivo culture in nude mice, biochemical studies demonstrated significantly higher levels of DNA, GAG, and collagen in the constructs that had first been matured in the bioreactor.
Other culture strategies have also gained momentum in efforts to meet the biochemical and biomechanical ideals of the prototype septal cartilage construct. Mechanical loading to cell-seeded constructs in the form of direct compression, specifically, has shown promise in its potential to increase cellular proliferation and ECM production over static controls. 61 , 62 , 63 , 64 , 65 Sah et al demonstrated increased protein production in dynamically compressed calf articular explants compared to controls. 66 Muack et al have subsequently demonstrated that bovine articular chondrocytes cultured in agarose hydrogels with application of dynamic loading express significantly more collagen and GAG than nonloaded counterparts. 64 The biomechanical properties of compressed constructs have been noted to be significantly enhanced as well. Bian et al evaluated adult canine chondrocytes seeded in agarose hydrogel that were subjected to dynamic loading versus free-swelling conditions. Results demonstrated that constructs with dynamic loading exhibited significantly greater stiffness than nonloaded constructs. Stiffness of dynamically loaded constructs compared favorably with (and exceed in some cases) that of native canine articular cartilage. 65
The mechanism by which mechanical compression can improve ECM may be multifactorial. Prior work by Takahashi et al demonstrated a two- to threefold increase in type II collagen expression in murine embryonic limb bud cells cultured in a collagen gel matrix with and without compressive forces. 67 Reverse transcription-polymerase chain reaction (RT-PCR) analysis revealed an increased level of Sox9, a transcriptional activator of type II collagen, and concurrent downregulation of interleukin 1-beta, a transcriptional repressor of type II collagen. It can also be hypothesized that direct compression reduces the water content of cartilage constructs and thereby increases concentration of extracellular components, thus strengthening the construct. This is consistent with the concept that typical cell-based engineered constructs tend to be highly hydrated. 68 Further study is needed to better elucidate the mechanosensitive properties of chondrocytes and the role of compaction in cartilage tissue engineering.
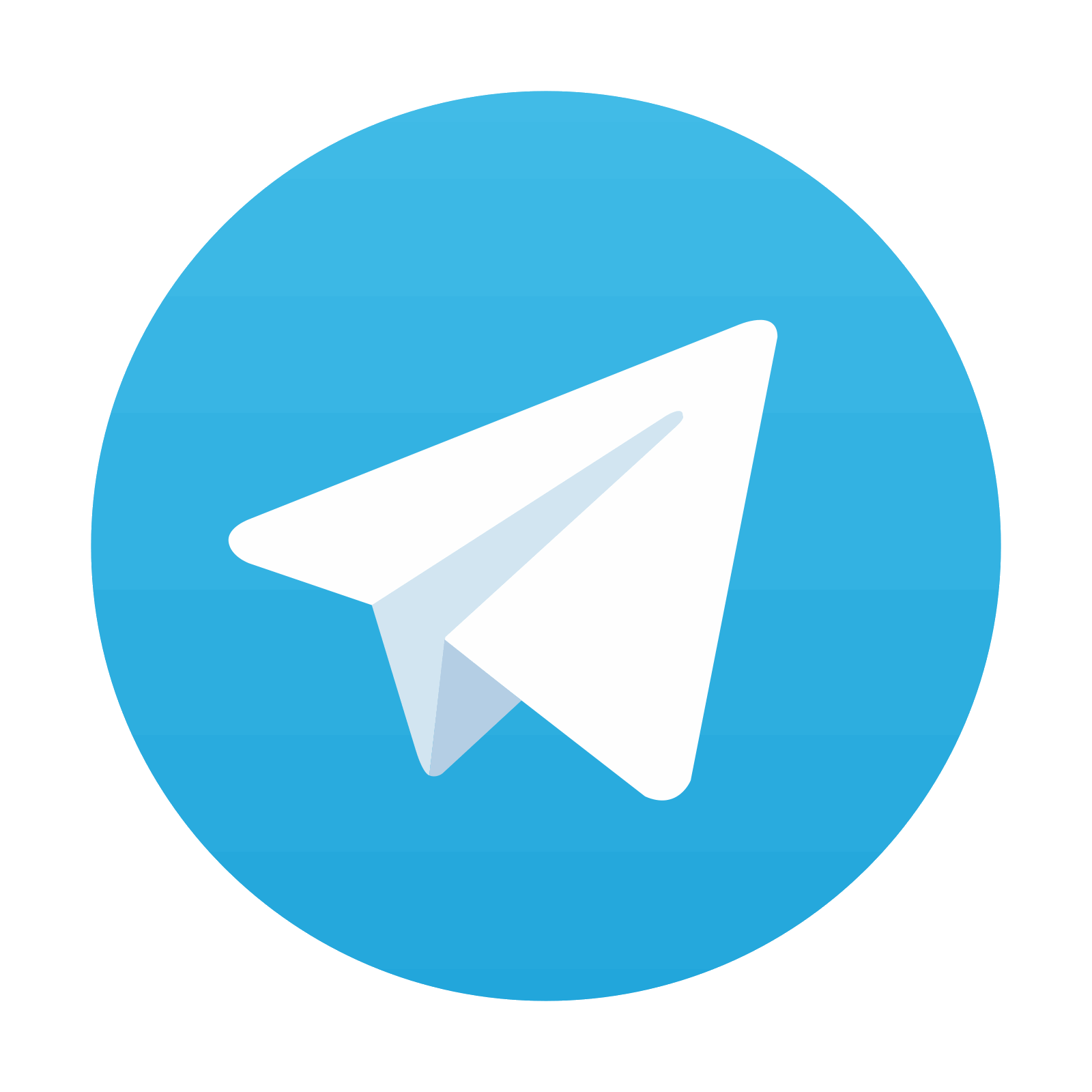
Stay updated, free articles. Join our Telegram channel
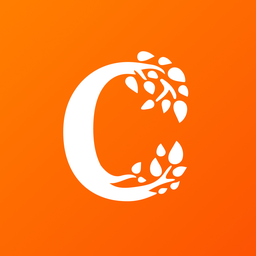
Full access? Get Clinical Tree
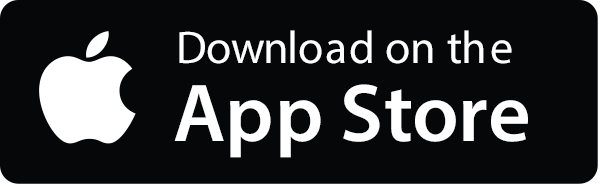
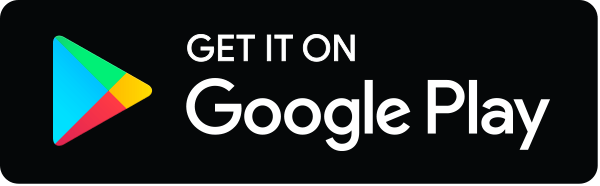
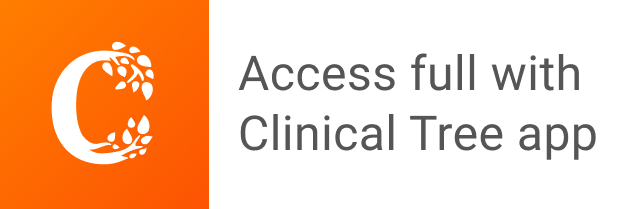