Figure 3-1 Schematic overview of embryonic development of human skin. (Courtesy of Dr. Michael Ioffreda.)
Figure 3-2 Histology of epidermis and dermis of 18-week normal human fetal skin (1-μm-thick, plastic-embedded section).
Figure 3-3 Schematic overview of the architecture and cytologic constituents of normal human skin. This projection demonstrates the cellular components of the epidermis and superficial dermis in greater detail, with epidermal strata denoted numerically. 1, stratum basalis; 2, stratum spinosum; 3, stratum granulosum; 4, stratum corneum. AC, eccrine acrosyringium; AP, arrector pili muscle; B, follicular bulb; DC, perivascular dendritic cell; E, epidermis; EC, coil of eccrine gland; ED, dermal eccrine duct; EN, endothelial cell; F, fibroblast; IN, follicular infundibulum; IS, follicular isthmus; LC, Langerhans cell; MC, mast cell; Mϕ, macrophage; PD, papillary dermis; RD, reticular dermis; S, sebaceous gland; SF, subcutaneous fat; V, vessel. (Courtesy of Dr. Michael Ioffreda.)
Figure 3-4 Histology of normal human skin. From top to bottom: epidermis, dermis, and upper part of the subcutaneous tissue. Note how the adipose tissue from the subcutis follows and surrounds adnexal structures within the dermis.
Figure 3-5 Histology of normal epidermis and superficial dermis. Note the separation of the delicate papillary dermal collagen from the underlying coarser reticular dermis by the horizontally aligned blood vessel representing a portion of the superficial vascular plexus.
The epidermal layer is composed primarily of keratinocytes (greater than 90%), with minority populations of Langerhans cells, melanocytes, neuroendocrine (Merkel) cells, and unmyelinated axons. In addition, the epidermis also contains specialized cuboidal epithelium that forms the spiraling acrosyringia of eccrine sweat ducts, as well as occasional cells putatively programmed for possible adnexal/glandular differentiation (e.g., Toker cells, as found in nipple epidermis in approximately 10% of individuals) (2,3). Architecturally, the epidermal layer has an undulant undersurface in two-dimensional sections, with downward invaginations termed retes and interdigitating mesenchymal cones termed dermal papillae (not to be confused with follicular papillae of the hair bulb). In reality, three-dimensional reconstruction reveals epidermal rete to form a honeycomb of interconnected ridges, with dermal papillae representing rounded conical invaginations not dissimilar to the undersurface of an egg carton. Separated from the epidermis by a structurally and chemically complex basement membrane zone, the dermis consists of endothelial and neural cells and supporting elements, fibroblasts, dendritic and nondendritic monocyte/macrophages, factor XIIIa–expressing dermal dendrocytes, and mast cells enveloped within a matrix of collagen and glycosaminoglycan (Table 3-1). Adnexae extend from the epidermis into the dermis and consist of specialized cells for hair growth, epithelial renewal (stem cells), and temperature regulation. Adnexal epithelium also appears to provide a safe haven for certain precursor cells (e.g., for melanocytes and dendritic cells) in a tissue niche sequestered from deleterious environmental influences at the skin surface. The subcutis is an underlying cushion formed by cells engorged with lipid and nourished by vessels that grow within thin intervening septae. The developmental anatomy of skin is important not only for understanding the basis for mature structural–functional relationships, but also in relation to certain skin tumors that recapitulate embryologic cutaneous structure.
EPIDERMIS
The epidermis comprises the following cell types:
• Keratinocytes
• Melanocytes
• Langerhans cells
• Merkel cells
Keratinocytes
Embryology
The development of in utero skin biopsy techniques gives practical importance to understanding the normal evolutionary histology of fetal skin. The epidermis begins as a single layer of ectodermal cells (4,5). By 5 weeks of gestational age, this has differentiated at least focally into two layers—the basal layer or stratum germinativum and the overlying periderm; by 10 weeks, an intervening layer—the stratum intermedium—develops (Fig. 3-1). Cells forming the periderm are large, bulge from the epidermal surface, and are bathed in amniotic fluid. By 19 weeks, there are several layers of intermediate cells, and the periderm has begun to flatten (Figs. 3-1 and 3-2). Keratinization is well developed by 23 weeks within the stratum intermedium in association with small keratohyaline granules. At this juncture, most of the periderm cells have shed, and the keratinizing cells that remain beneath represent the newly formed stratum corneum (5).
By ultrastructure, the histogenesis of the epidermis involves early formation of immature desmosomes and early hemidesmosomes and distinct basement membrane (6 to 7 weeks of gestational age) (6,7). Anchoring filaments are observed several weeks later, and the basement membrane appears structurally mature by the end of the first trimester. The surface cells of the periderm display numerous microvilli and cytoplasmic microvesicles, increasing the area in contact with amniotic fluid and suggesting active interchange between the periderm cells and the amniotic fluid (4). Tonofilaments are sparse until 16 weeks, when dense accumulations are observed in cells of the intermediate layer as evidence of beginning keratinization (5).
Normal Microanatomy
The keratinocytes differ from the dendritic or clear cells (melanocytes and Langerhans cells) by their larger size, an ample amount of stainable cytoplasm, and the presence of intercellular bridges. As they differentiate into horny cells, the keratinocytes are arranged in four layers from bottom to top (Figs. 3-6 to 3-12):
• Basal cell layer (stratum basalis)
• Squamous cell layer (stratum spinosum)
• Granular layer (stratum granulosum)
• Horny layer (stratum corneum)
Figure 3-6 Histology of normally anucleate stratum corneum, the terminal differentiation product of underlying basal and suprabasal cells. Note the characteristic “basket-weave” architecture typical of nonacral sites.
Figure 3-7 Histology of normal acral skin illustrating the thick and compact stratum corneum and the presence of a stratum lucidum (gray blue) in the lower portion of the horny layer.
Figure 3-8 Ultrastructure of stratum corneum showing transition of intracellular tonofilaments within viable cells to anucleate stratum corneum at the epidermal surface. The darker granules directly beneath the stratum corneum represent keratohyaline granules.
Figure 3-9 Evidence of basal cell layer replicative potential by Ki-67 immunostaining. Note the predominance of labeled cells in the basal cell layer of the epidermis and the included portion of a follicular infundibulum.
Figure 3-10 Mucosal epithelium of the oral cavity showing stratified squamous epithelium without a well-formed granular or cornified layer. Note the cytoplasmic pallor due to glycogenation and delicate underlying connective tissue.
Figure 3-11 Ultrastructure of a desmosome. Note the two electrodense plaques, each located within the cytoplasm of the two keratinocytes that are connected by the desmosome.
Figure 3-12 Immunohistochemistry for a pan-keratin (including low- and high-molecular-weight keratins) showing diffuse expression in the epidermis and adnexal structures. Inset: Preferential expression of keratin 14 by the basal and parabasal layers (immunofluorescence).
An additional layer: Stratum lucidum can be recognized on palms and soles (see Regional Variations) (Fig. 3-7).
Stratum Basalis
The basal cells form a single layer, are ovoid in contour, and lie with their long axes perpendicular to the underlying basement membrane. They have a more basophilic cytoplasm than cells of the stratum spinosum, often contain melanin pigment transferred from adjacent melanocytes, and contain dark-staining round to oval nuclei (Fig. 3-5). They are connected with each other and with the overlying squamous cells by intercellular bridges united by desmosomes (discussed in detail later). At their base, the basal cells are attached to the subepidermal basement membrane zone by modified desmosomes, termed hemidesmosomes. Basal cells and overlying squamous cells contain keratin intermediate filaments termed tonofilaments, which form the developing cytoskeleton; these cells and related cytoskeletal proteins ultimately give rise to the anucleate stratum corneum at the epidermal surface (Figs. 3-6 and 3-8).
Most of the mitotic activity in normal human epidermis occurs in the basal cell layer (8). By tritiated-thymidine labeling of cells in the S phase of DNA synthesis, which is approximately seven times longer than the mitotic or M phase, many more tritiated-thymidine-labeled basal cells can be visualized than mitotically active basal cells. Ki-67 labeling is a useful way of determining cycling cells in paraffin sections, and, as with tritiated thymidine, the vast majority of labeled cells in normal skin are within the basal cell layer of the epidermis (Fig. 3-9).
Stratum Spinosum
The polyhedral cells of the stratum spinosum overlying the basal cell layer form a mosaic usually 5 to 10 layers thick. They become progressively flattened toward the skin surface (Fig. 3-6). The cells are separated by spaces that are traversed by intercellular bridges united by desmosomes. These intercellular spaces contain neutral mucopolysaccharides and acid mucopolysaccharides (glycosaminoglycans). Hyaluronic acid, an important component of the glycosaminoglycans, is quite abundant in the matrix between keratinocytes, occurring predominantly in the spinous layers but also in the basal layer and the stratum corneum (9). The intercellular cement substance between two adjacent keratinocytes, referred to also as glycocalyx, has a gel-like consistency. On the one hand, it provides cohesion between the epidermal cells, and on the other hand, it allows the rapid passage of water-soluble substances through the intercellular spaces; furthermore, it allows the opening up of desmosomes and individual cell movement (10–12).
The tonofilaments within the cytoplasm of the keratinocytes of the stratum spinosum are loose bundles of electron-dense filaments, each filament measuring 7 to 8 nm in diameter. These structures correlate with keratin proteins, as may be demonstrated by immunohistochemistry (see later discussion on immunohistochemistry) (Fig. 3-12).
The tonofilaments at one end are attached to the attachment plaque of a desmosome, and the other end of the tonofilaments form the cytoskeleton within the cytoplasm. Electron microscopy shows that the intercellular junctions (desmosomes) consist of two dense plaques 10 to 15 nm thick on adjacent cell membranes. A 30-nm-wide multilayered zone is present between them (Fig. 3-11). The binding of adjacent keratinocytes via interaction of these ultrastructurally complex adjacent desmosomal plaques (10,11) has recently been understood at a molecular level (Fig. 3-11; see also Fig. 3-23). A key family of molecules is the cadherins, derived from multiple genes and representing Ca2+-dependent cell adhesion molecules with a characteristic single-spanning transmembrane structure (13). Desmosomal cadherins are desmogleins and desmocollins (14) that localize to desmosomes and are linked to intracytoplasmic intermediate filaments by plakoglobin and desmoplakin. Within the desmosome complex, desmogleins within the cell membrane bind to plakoglobin through their cytoplasmic domain. Intermediate keratin filaments anchor at the desmosomal plaque, possibly by way of the carboxy-terminal domain of plakoglobin. The structural importance of these molecules in uniting keratinocyte cytoskeletons within the epidermis is indicated by the disorders pemphigus vulgaris and pemphigus foliaceus, in which autoantibodies to desmoglein 3 and desmoglein 1, respectively, result in clinical blisters due to loss of cell–cell adherence (Fig. 3-23) (see Chapter 9) (15,16). Desmoglein 3 is normally concentrated between immediately suprabasal keratinocytes, whereas desmoglein 1 is distributed preferentially among keratinocytes directly beneath the stratum corneum. Indeed, a remarkable correlation has been established between desmoglein expression in normal skin and pathologic lesions in which specific desmogleins are disrupted with regard to the plane of blister formation due to loss of cell–cell adhesion.
Stratum Granulosum
The cells of the granular cell layer are flattened, and their cytoplasm is filled with keratohyaline granules that are deeply basophilic and irregular in size and shape (Fig. 3-6). The thickness of the granular layer in normal skin is generally proportional to the thickness of the horny layer: It is only one to three cell layers thick in areas in which the horny layer is thin but measures up to 10 layers in areas with a thick, horny layer, such as the palms and soles (Figs. 3-5 and 3-7). In the process of keratinization, the keratohyaline granules form two structures: the interfibrillary matrix or filaggrin, which cements the keratin filaments together, and the inner lining of the horny cells, the so-called marginal band. Whereas the tonofibrils contain only small amounts of sulfur as sulfhydryl groups, the interfibrillary matrix and the marginal band contain about 10 times the amount of sulfur that is present in the tonofibrils, predominantly as disulfide bonds of cystine (17,18). Consequently, the tonofibrils are soft and flexible, whereas the matrix and marginal band provide necessary strength and stability (18). Thus, the keratin of the epidermis represents “soft” keratin, in contrast to the “hard” keratin of the hair and nails, in which keratohyaline granules are lacking and the tonofibrils themselves harden through the incorporation of disulfide bonds (19). “Soft” keratin desquamates as the result of enzymatic action, but the “hard” keratin of the hair and nails does not and thus requires periodic cutting.
The granular cell layer represents the mature keratin-forming transitional zone of the epidermis, in which the dissolution of the nucleus and other cell organelles is prepared. In contrast to the stratum basalis and stratum spinosum, in which lysosomal enzymes, such as acid phosphatase and aryl sulfatase, are present as only a few granular aggregates, there is diffuse staining for lysosomal enzymes in the granular cell layer. These diffusely staining lysosomal enzymes probably play an important role in the autolytic changes occurring in the granular layer (20).
Stratum Corneum
Unlike the nucleated cells of the other epidermal layers, the cell remnants of the normal stratum corneum are anucleate. Thus the normal horny layer stains eosinophilic. The thickness of the horny layer is often difficult to ascertain in formalin-fixed specimens because the outer cell layers may become detached. The portion of the horny cytoplasm that contains disulfide bonds of cystine tends to shrink on formalin fixation to form a shell along the cell membrane, resulting in the characteristic basket-weave architecture in routine sections (Fig. 3-6) (21). In contrast, glutaraldehyde fixation used for electron microscopy causes precipitation of the formalin-soluble substances within the horny cells and allows staining of the contents of the horny cells with reagents such as uranyl acetate and lead citrate. With a fluorescent stain, it can be shown that the cells of the horny layer are arranged in orderly vertical stacks (22).
Maturation and Terminal Differentiation
Maturation of keratinocytes from basal cells to the horny layer normally takes approximately 30 days, although this may be radically altered in certain disease states. The process involves transition from ovoid basal cells to polyhedral cells in squamous cell layer, to more flattened cells associated with acquisition of keratohyaline granules, and finally to fully keratinized cells without nuclei in horny layer. The transformation of granular cells into horny cells usually is abrupt. The keratinization process during maturation involves three intracellular organelles:
• tonofilaments in stratum basalis
• keratohyaline granules in stratum granulosum
• membrane-coating granules (Odland bodies) in stratum corneum
Tonofilaments are approximately 8 nm thick, and they are present throughout the epidermis, although they become increasingly prominent as they approach the epidermal surface. By electron microscopy, the cytoplasm of the cells in the lower portion of the horny layer shows relatively electron-lucent tonofilaments, embedded in an interfilamentous substance (Fig. 3-8) (23). In the upper portions of the horny layer, however, the cells lose their filamentous structure. Together with the sudden keratinization of the horny cells, an electron-dense, homogeneous marginal band forms in their peripheral cytoplasm in close approximation to the trilaminar plasma membrane. In the lowermost horny layer, the trilaminar plasma membrane is preserved outside of the marginal band; in the midportion of the horny layer, it becomes discontinuous and then desquamates, so that the marginal band serves as the real cell membrane (24).
Keratohyaline granules are biochemically complex and composed of profilaggrin, loricrin, and keratin filaments. Profilaggrin is a filaggrin precursor, which is a histidine-rich protein (25). When granular cells are converted to cornified cells, the filaggrin precursor is broken down into many units of filaggrin. Immunohistochemistry reveals that involucrin, a structural component of mature squamous epithelium, is incorporated into the marginal band as part of the formation of the protein envelope that characterizes squamous cells immediately prior to terminal differentiation (26). Desmosomal contacts are at first still present in the horny layer but disappear before desquamation of the horny cells.
Odland bodies, also called membrane-coating granules, lamellar granules, or keratinosomes are small organelles that are discharged from the granular cells into the intercellular space and that have two important functions: they establish a barrier to water loss, and they mediate stratum corneum cell cohesion. Lamellar granules appear first in the perinuclear cytoplasm in the stratum spinosum. Higher up in the epidermis, they rapidly increase in number and size (27). Both within and outside the granular cells, lamellar granules are round or oval, measure approximately 300 to 500 nm in diameter, and, by electron microscopy, possess a trilaminar membrane and a laminated interior. During maturation, the lamellar granules fuse with the plasma membrane of a granular cell, secreting its contents into the intercellular spaces, an event associated with establishing a permeability barrier near the epidermal surface (see below) (28).
Regional Variation
Skin shows considerable variation among different body sites, and appreciation of such normal differences is critical to accurate diagnostic assessment. Perhaps the most profound differences exist at mucosal and paramucosal sites. For example, with the exception of the dorsum of the tongue and the hard palate, the mucous membrane of the mouth possesses neither a granular nor a horny layer (Fig. 3-10). When these layers are absent, the epithelial cells may appear vacuolated, largely as a result of their glycogen content. Electron microscopic examination reveals that the number of tonofilaments diminishes in the upper layers, and they become dispersed. Large aggregates of glycogen are present in the cells. The epithelial cells of the oral mucosa show only few well-developed desmosomes. Instead, they show numerous microvilli at their borders. They are held together by an amorphous, moderately electron-dense intercellular cement substance, the dissolution of which causes the detachment of the uppermost cells (24).
In contrast to mucosal epithelium, acral skin has a thick stratum granulosum and stratum corneum. Stratum lucidum forming the lowest portion of the horny layer can be best recognized in acral skin, especially on the palms and soles (Fig. 3-7).
Specialized Structure and Function
Superficial Cellular Cohesion
The number of tonofilaments in the epidermis increases in the upper portion of the squamous layer. The earliest formation of keratohyaline granules consists of the aggregation of electron-dense ribonucleoprotein particles largely along tonofilaments. The keratohyaline granules increase in size through peripheral aggregation of ribonucleoprotein particles, and they surround more and more tonofilaments (29). By extending along numerous tonofilaments, the keratohyaline granules assume an irregular, often star-shaped outline and may reach a size of 1 to 2 μm. After ultimately ensheathing all tonofilaments, they form in the horny cells the electron-dense interfilamentous protein matrix of mature epidermal keratin. Profilaggrin in keratohyaline granules at the level of the stratum granulosum breaks down into many units of filaggrin in the stratum corneum, where filaggrin then aggregates with keratin filaments and acts as “glue” for keratin filaments. Recent studies indicate a flaggrin gene mutation occurring in up to 10% of the European population, an event associated with increased risk of developing atopic dermatitis with asthma (30), as well as ichthyosis vulgaris (31).
Barrier Function
Odland granules fuse and completely fill the intercellular spaces at the level of the granular layer, thus to establish a barrier to water loss. They contain neutral sugars linked to lipids and/or proteins; hydrolytic enzymes, possibly charged with degrading intercellular materials; and free sterols. The stratum granulosum interstices contain free sterols and sugars; the stratum corneum intercellular spaces stain as a pure neutral lipid mixture with abundant free sterols but no sugars (28). Sugars are cleaved at the granular-cornified layer interface by sugar-specific glycosidases. The lipids formed in these organelles act as hydrophobic material, which is important to the barrier function (32,33). A similar permeability barrier exists in the oral mucosa. Lamellar granules may also contribute to cohesion between the cells of the lower stratum corneum as a result of the lipids that they contain. The action of enzymes, such as steroid sulfatase, removes the lipids from the upper stratum corneum and brings about desquamation of the cells there (34).
Enzyme Activity
Primary lysosomes that are membrane bound and contain a variety of hydrolytic enzymes, such as acid phosphatase, aryl sulfatase, and β-galactosidase, are seen in small numbers within keratinocytes, largely but not exclusively in the basal cell layer and lower squamous cell layer (12). A great number of lysosomal enzymes, primarily located free within the cytoplasm, are demonstrable in the granular layer and in the lowermost horny layer. Lysosomal enzymes are found also in the lamellar granules and are located within granular cells and after their discharge into the intercellular space (35). Secondary lysosomes, also called phagolysosomes, are present in the lower epidermis, especially in the basal cells, where they digest phagocytized melanosomes, usually as melanosome complexes (see later discussion). In cases of epidermal injury, such as sunburn or interface dermatitis, numerous phagosomes are observed that contain remnants of organelles from damaged cells (35).
Immune Functions of Keratinocytes
In recent years, the epidermal keratinocyte has been recognized as a potent source of immunogenic molecules. Keratinocytes are capable of producing interleukins (ILs) (IL-1α, IL-1β, IL-6, IL-8), colony-stimulating factors (CSFs) (IL-3, granulocyte-macrophage-CSF, granulocyte-CSF, macrophage-CSF), interferons (IFNs) (IFNα, IFNβ), tumor necrosis factors (TNFs), transforming growth factors (TGFs) (TGFα, TGFβ), and growth factors (platelet-derived growth factor, fibroblast growth factor, melanocyte-stimulating hormone) (36). Some of these substances are expressed constitutively, whereas others are synthesized only after signal transduction initiated by external or systemic cues (37). Accordingly, keratinocytes may play an active role in elaboration of molecular signals that facilitate lymphocyte homing and local activation, enabling certain dermal cells to mature and regulating synthesis of extracellular matrix molecules.
Immunohistochemistry
Immunohistochemical techniques have become an important part of the diagnostic arsenal of pathologists, and the expression of keratin by poorly differentiated tumor cells is considered an important marker of epithelial differentiation. Keratins consist of two families of intermediate filament proteins, with type I and type II members always occurring as pairs (38,39). In humans, keratin genes are clustered as families in two regions of the genome, the type I genes on chromosome 17q21.2 and those encoding type II keratins on chromosome 12q13.13. There are 54 functional keratin genes, 27 in each of these domains (39). Different epithelia express different keratins. In addition, different keratins are expressed by the different layers of the human epidermis, reflecting the variable degree of differentiation in keratinocytes. Keratin 5 (K5) and keratin 14 (K14) are predominantly expressed by basal cells (40) (Fig. 3-12). When these cells differentiate, they downregulate expression of mRNAs encoding these two keratins and induce expression of new sets of keratins specific for individual programs of epithelial differentiation (Fig. 3-12). Moreover, unlike other basal cells that express K14, basal cells in the general region where stem cells reside also express keratin 15, indicating that biosynthetic heterogeneity exists within the basal layer with regard to expression of cytoskeletal elements (41,42). Suprabasal keratinocytes predominantly express keratin 1 and keratin 10. Keratin 2 is expressed by terminally differentiated keratinocytes. Keratin 9 is expressed by suprabasal keratinocytes in palmar and plantar epidermis. The pattern and type of keratin expression is different in the epidermis and adnexal structures. It is important to be familiar with the expression of keratin by normal structures because specific mutations are associated with different conditions (e.g., epidermolysis bullosa simplex variants are associated with mutations in keratin 5 and 14 genes, whereas mutations for keratin 1 and 10 genes are associated with bullous ichthyosiform erythroderma; mutations in the keratin 2 gene result in bullous ichthyosis of Seimens, and mutations of keratin 9 genes are responsible for epidermolytic palmoplantar keratoderma) (Table 3-2).
A more novel epithelial immunohistochemical marker is p63, a member of the p53 gene family implicated in both the development and maintenance of stratified epithelial tissues, including the epidermis. Increasing data support p63 function in the regenerative capacity of basal keratinocytes by maintaining cell proliferation (43) as well as inducing epidermal commitment of embryonic stem cells (44,45). The critical contribution of p63 to embryonic development is underscored by the severe abnormalities seen at birth in mice null for p63, including lack of epidermis. Mutations in p63 have been linked to ectodermal dysplasia syndromes with a skin phenotype, indicating the relevance of p63 to normal epidermal development in humans (46). In normal human epidermis, in hair follicles, and in stratified epidermal cultures, p63 protein is principally restricted to cells with high proliferative potential and is absent from the cells that are undergoing terminal differentiation. Accordingly, p63 expression is seen in basal/suprabasal cells of the epidermis, outer root sheath, and hair matrix cells of the hair follicle, basal cells situated in the outermost layer of sebaceous glands, and outer layer cells of the ductal portion and myoepithelial cells of the secretory portion of the sweat glands (Fig. 3-13). p63 is overexpressed in the vast majority of squamous cell carcinomas and basal cell carcinomas, and appears to be an especially helpful marker for poorly differentiated squamous cell carcinomas, including the sarcomatoid variant (47).
Figure 3-13 p63 immunostain shows intense nuclear positivity in all epithelial compartments, including the epidermis and adnexal structures. The staining is more intense in basal and parabasal areas.
Melanocytes
Embryology
The appearance of melanocytes in the epidermis takes place in a craniocaudal direction, in accordance with the development of the neural crest, from which the melanocytes are derived. By enzyme histochemistry, melanocytes can be identified in the epidermis of the head region during the latter part of the third fetal month; in the more caudal body regions, the earliest-formed melanin can be observed only in the latter part of the fourth month. Because melanocytes are functionally immature during their migration through the fetal dermis, they cannot be identified by histochemical methods until they have reached the epidermis (48). Electron microscopy and immunohistochemical approaches (e.g., stain for HMB-45 protein) allow for an earlier recognition of melanocytes in the epidermis than is possible by light microscopy (49,50).
The tyrosine kinase receptor KIT and its ligand stem cell factor play a critical role in melanocyte physiology, influencing migration, proliferation, differentiation, and survival (51–53). Piebaldism, a genetic disorder, results from KIT receptor defects secondary to mutations of the c-kit proto-oncogene. Patients with piebaldism present at birth with white patches of skin and hair that lack melanocytes, supporting the major role of KIT in melanocyte migration from the neural crest to the skin (54).
Microphthalmia transcription factor (MITF) is a nuclear protein involved in the embryonic development of melanocytes and the regulation of transcription of genes involved in melanin synthesis, such as tyrosinase. The microphthalmia (mi) gene is located on chromosome 3p. Since its discovery as a mutant allele producing mice devoid of viable melanocytes, mi has emerged as the gene whose mutation is responsible for the human pigmentation condition Waardenburg syndrome IIa, as well as a variety of cellular defects involving retinal pigment epithelium, osteoclasts, and mast cells (55).
Normal Microanatomy
Mature melanocytes are melanin-synthesizing dendritic cells located within the basal layer of the epidermis, hair bulb, and outer root sheath of hair follicles (Figs. 3-14 to 3-17). In sections stained with hematoxylin–eosin, the dendrites of epidermal melanocytes are usually not seen, and the cell bodies are randomly dispersed within the basal cell layer. They contain round to oval, dark-stained nuclei that are generally smaller than those of basal keratinocytes and what may appear as a clear halo of surrounding cytoplasm, largely as the result of shrinkage during tissue processing. Although the number of melanocytes in relation to basal cells varies with the body region and increases with repeated exposure to ultraviolet light, the average number of clear cells in hematoxylin–eosin-stained vertical sections is 1 of 10 cells in the basal layer, and a similar ratio was found with immunohistochemical studies (56,57). Melanin is transferred by means of the dendritic processes (Figs. 3-14 and 3-15A, B) from the melanocytes to the basal keratinocytes, where it is first stored and later degraded. As a rule, a greater amount of melanin is present in the basal keratinocytes than in the melanocytes, and often basal cells at the tips of rete ridges are preferentially more melanized. Because only about 10% of the cells in the basal layer are melanocytes, each melanocyte supplies several keratinocytes with melanin, forming with them an epidermal-melanin unit (58).
Figure 3-14 S100 immunostain is expressed by junctional melanocytes and also by Langerhans cells in the mid-epidermis and dermis.
Figure 3-15 Melan-A/MART-1 immunostain demonstrates normal melanocytes within the epidermal basal layer. This is a more specific melanocytic marker compared to S100 (A). Note the dendritic extension at higher magnification (B). This melanocyte appears to be suprabasal as a result of the tangential plane of the section.
Figure 3-16 Ultrastructure of melanocytes. These cells characteristically “hang down” from the basal cell layer and are devoid of tonofilaments of desmosomes (A). They contain characteristic melanosomes in varying stages of melanization (B).
Figure 3-17 A: Melanocytes in sun-damaged skin may be increased in number and size as depicted in this microphotograph. Note the marked dermal solar elastosis. B: MART-1 immunostain highlights an increase in melanocyte density in sun-damaged skin.
In persons with a light skin color, staining with hematoxylin–eosin may reveal few or no melanin granules in the basal cell layer. In persons with a dark skin color, especially individuals of African heritage, melanin granules are present in the basal cell layer, as well as throughout the epidermis, including the horny layer, and in some instances in the upper dermis within macrophages, called melanophages. Accordingly, ethnic background and body site are critical in separating normal variations from true pathology.
Special Stains
Melanocytes may be difficult to appreciate on hematoxylin–eosin-stained sections, and there are several special stains that can facilitate their detection by light microscopy. Silver stains indicate the presence of melanin, which is both argyrophilic and argentaffin. Argyrophilia is based on the ability of melanin to be impregnated with silver nitrate solutions, which, on reduction with hydroquinone to silver, stain black. Because melanin is argentaffin, ammoniated silver nitrate may be reduced by phenolic groups in the melanin, forming black silver precipitate (e.g., the Fontana-Masson method). Neither of these methods is entirely specific for melanin, however. Melanin may be bleached by a strong oxidizing agent, such as hydrogen peroxide or potassium permanganate, a method that permits more specific identification (59) and is used in heavily melanized tumors, where pigment may obscure nuclear detail. The dihydroxyphenylalanine (dopa) reaction, which stains melanocytes dark brown to black, imitates physiologic melanin formation, which begins by tyrosinase-dependent hydroxylation of tyrosine to dopa and the oxidation of dopa to dopa-quinone, which is subsequently polymerized into melanin. Thus, the dopa reaction is in essence an assay for tyrosinase activity in melanosomes (59).
The use of immunohistochemical techniques for labeling melanocytes is becoming increasingly important, and several melanocytic markers that work in paraffin-embedded tissues are available (Table 3-3). Immunohistochemical detection of melanocytes has been classically and most commonly accomplished by polyclonal antibodies to S100 protein. The antigen S100, a family of calcium-binding proteins (molecular weight 21,000), originally isolated from bovine brain extract (60), is present in the cytoplasm and nucleus of a variety of cells, including those of neural melanocytic lineage (61). The function of S100 within melanocytes is unclear, although different subtypes of S100 appear to have different functions in different cell types. The member of the S100 family predominantly expressed in normal melanocytes is S100B (62), whereas S100A subtypes have been reported in various skin lesions. In the skin, in addition to melanocytes, S100 labels Langerhans cells, specialized macrophages, Schwann cells, sweat glands, and adipocytes, and therefore, although it is a sensitive marker, it lacks specificity (Fig. 3-14; see also Figs. 3-35F, 3-52C, 3-53A, and 3-54B).
The search for new targets for immunotherapy in patients with melanoma has generated several novel reagents valuable for diagnostic pathology. By using cytotoxic T-cell assays, various antigens that are recognized by autologous cytotoxic T lymphocytes were identified (63). A group of these antigens is referred to as melanocyte differentiation antigens because their expression is restricted to cells of melanocytic lineage. A number of reagents to melanocyte differentiation antigens suitable for analysis of paraffin-embedded material have become available, including the classically used monoclonal antibody HMB-45 and additional reagents such as monoclonal antibodies to Melan-A/MART-1 (64,65) (Fig. 3-15), tyrosinase, and PNL2. HMB-45 antibody reacts with a cytoplasmic epitope, the premelanosome glycoprotein gp100 (75 to 100 kD), in cells of most but not all melanomas and in a minority of nevi (66,67). HMB-45 is negative in normal epidermal melanocytes in adults; however, it is expressed by melanocytes in fetal skin and stimulated adult melanocytes and is considered a highly specific antibody for the immunocytochemical substantiation of the diagnosis of malignant melanoma.
The advantage of the newer melanocyte differentiation antigens (e.g., Melan-A/MART-1) is a stronger sensitivity when dealing with melanocytic neoplasms compared to HMB-45 and stronger specificity when compared with S100. The gene for Melan-A/MART-1 was independently cloned by two groups of scientists, which explains the two different designations (MART stands for Melanoma Antigen Recognized by T cells) (68). This melanocytic differentiation antigen is a small cytoplasmic protein (22 kD) with distinctive nucleotide sequences and genetic organization that is believed to be a melanosomal component. Tyrosinase is a key enzyme in melanin biosynthesis and represents a marker of melanocytic differentiation. T311, a murine monoclonal antibody to tyrosinase, is a reliable marker of melanocytes in paraffin-embedded sections (69). PNL2 is a monoclonal antibody directed against a fixative-resistant melanocyte antigen. The exact nature of the antigen recognized by PNL2 remains to be defined. The analysis of PNL2 immunostaining on a broad range of normal or malignant human tissues and on various melanocytic lesions revealed its high specificity (70). Despite the advent of these new markers, S100 and HMB-45 remain very important tools when dealing with tumors, and S100 remains the more sensitive (although not very specific marker) for neoplastic melanocytes, especially spindle cell lesions (e.g., desmoplastic melanoma).
MITF, another novel melanocyte differentiation marker, was shown to be less sensitive and not restricted to melanocytes. D5 (monoclonal antibody to MITF) was generated from the consensus region shared by various isoforms of MITF. Only one isoform, MITF-M, is melanocyte specific. Other isoforms that are recognized by D5 can be found in various nonmelanocytic cell types (Table 3-3) (71).
Ultrastructure
Melanocytes differ from keratinocytes by possessing no tonofilaments or desmosomes (Fig. 3-16A). At their base, where they lie in close apposition to the lamina densa, melanocytes show structures resembling, yet smaller than, hemidesmosomes of basal keratinocytes (72). Melanosomes are the most characteristic organelles of the melanocyte, and identification of various stages of their formation within a cell assists in its ultrastructural identification as a melanocyte (in contrast to keratinocytes that contain via transfer only fully melanized organelles) (Fig. 3-16B). Melanosomes are membrane-bound organelles that in their development from stage I to stage IV gradually move from the cytoplasm of the melanocyte into the dendritic processes. As melanosomes mature, their content of melanin increases, and their concentration of melanogenic enzyme decreases (73).
Stage I melanosomes are round, 0.3 μm in diameter, and contain no melanin (74). Stage II–IV melanosomes are ellipsoid and measure approximately 0.5 μm in length. They contain longitudinal filaments that are cross-linked with one another. Enzyme activity is present both on the enveloping membrane and on the filaments. Melanin deposition on the cross-linked filaments begins at stage II. Stage III melanosomes have only little tyrosinase activity but show continued melanin deposition, partially through nonenzymatic polymerization. Stage IV melanosomes are fully melanized and no longer possess tyrosinase activity. Melanin, which is homogeneous and electron dense, fills the entire organelle at this stage, obscuring its internal structure.
Regional and Racial Variation
The highest concentration of melanocytes is found on the face and the male genitals, about 2,000/mm2, and the lowest on the trunk, about 800/mm2 (75–77). No significant difference in the density of distribution of melanocytes for any given area of the skin exists between African American and Caucasoid skin. In the former, the melanocytes are uniformly highly enzymatically reactive, whereas the melanocytes of Caucasoids, when not exposed to sunlight, are highly variable in dopa reactivity (78). In addition, African American skin contains larger and more highly dendritic melanocytes than does Caucasoid skin (76,77). Finally, transferred melanosomes within keratinocytes of people of African descent tend to be individually distributed, whereas those in keratinocytes of Caucasians are packaged in membrane-enclosed aggregates (79–82). The melanocortin 1 receptor gene (MC1R) is responsible for normal pigment variation in humans and is highly polymorphic with numerous population-specific alleles. Some MC1R variants have been associated with skin cancer risk (82).
Melanocytes in Sun-Exposed Areas
Regional variation of melanocyte number and morphology may also be influenced by environmental factors, such as sun exposure. After a single exposure to ultraviolet light in vivo, the skin of Caucasoids, when examined with the dopa reaction, shows no increase in the density of the melanocyte population but does show an increase in the size and functional activity of the existing melanocytes (83). Repeated exposure to ultraviolet light, however, causes an increase in the concentration of dopa-positive melanocytes, as well as an increase in their size and functional activity (78,84). Thus, examination of habitually exposed and of unexposed skin from adjacent anatomic sites, such as the lateral and medial aspects of the upper arm, has shown a twofold-higher concentration of melanocytes in the former, and the melanocyte concentration is even higher in areas such as head and neck (85).
Surgical pathologists and dermatopathologists often face the difficulty of having to distinguish melanocyte hyperplasia of sun-damaged skin from melanoma in situ (e.g., when evaluating surgical margins in melanoma excisions). It is known that melanocytes increase in number and size in sun-damaged skin (Fig. 3-17A, B). On immunohistochemical studies, long-standing sun-exposed skin has been reported to have an average of 15 to 20 melanocytes per high-power field (in 0.5 mm of skin) (85,86), some degree of contiguous growth (up to 9 adjacent melanocytes), and melanocytes extending to hair follicles but not reaching the level of the uppermost portion of sebaceous glands. However, there should not be significant confluence, deep follicular penetration, nesting, or pagetoid spread.
Specialized Structure and Function
Melanogenesis
Enzymatic melanogenesis involves tyrosinase as the melanogenic enzyme (77,87). Tyrosinase is a copper-containing enzyme that catalyzes the hydroxylation of tyrosine to dopa and the oxidation of dopa to dopa-quinone. However, before tyrosinase can act on tyrosine, two cupric atoms present in tyrosinase must be reduced to cuprous atoms. It is believed that, in addition to being a substrate, dopa activates this reduction, thereby acting as a cofactor in the reaction. The conversion of tyrosine to melanin by tyrosinase is characterized by a variable lag period. When tyrosinase is present in low concentrations, as in epidermal melanocytes of nonirradiated skin, this lag period is markedly prolonged, and no use of tyrosine by tyrosinase is detectable. In contrast, in skin exposed in vivo to ultraviolet light, as well as in epidermal sheets and in hair bulbs, tyrosinase activity is detectable with tyrosine as substrate (58,77,88). Because there is no lag period with dopa as substrate, tyrosinase in epidermal melanocytes can be readily demonstrated even in nonirradiated skin when skin sections are incubated in dopa rather than in tyrosine. The enzyme acting on dopa is therefore thought to be tyrosinase rather than dopa-oxidase.
The melanogenic enzyme tyrosinase is synthesized in the Golgi-associated endoplasmic reticulum, in which tyrosinase condenses in membrane-limited vesicles. This process has been observed by electron microscopy in epidermal melanocytes after in vivo ultraviolet irradiation with either dopa or tyrosine as substrate (89). Subsequently, these tyrosinase units are transferred to dilated tubules of the smooth endoplasmic reticulum. There, tyrosinase is incorporated into a structural protein matrix containing filaments that have a distinctive periodicity. This then represents a stage I melanosome (90). Few stage I and II melanosomes have acid phosphatase activity, but the proportion of acid phosphatase–positive melanosomes increases in stage III, reaching a maximum in stage IV (91). This enzyme may play a role in the degradation or transfer of melanosomes. Detailed analysis of nonmelanosomal regulatory factors in melanogenesis has revealed coated vesicles to be richest in tyrosinase and catalase, whereas premelanosomes have the highest concentrations of peroxidase (92). Among relevant metal ions, premelanosomes contain higher amounts of copper, zinc, and iron than do coated vesicles.
Melanin Transfer
The transfer of melanosomes from melanocytes to epidermal keratinocytes and to hair cortex cells is the result of active phagocytosis of the tips of melanocytic dendrites by keratinocytes and hair cortex cells as demonstrated in tissue cultures (93) and in epidermal reconstructs seeded with melanocytes (94). Once incorporated into keratinocytes, melanosomes aggregate to form protective parasoles above the keratinocyte nuclei. As previously noted, melanin in Caucasoids is concentrated in basal keratinocytes, whereas in people of African ancestry, distribution is more diffuse within the epidermis (95,96). Both melanin formation and melanin transfer are enhanced by various stimuli, notably by skin exposure to ultraviolet (UV) light. Data suggest a central role for p53 in UV-induced skin melanization and potentially certain forms of pathologic hyperpigmentation (97). UV-induced melanization, or sun tanning, requires the induction of α-melanocyte-stimulating hormone (α-MSH) secretion by epidermal keratinocytes. This phenomenon requires α-MSH generation as a cleavage product of pro-opiomelanocortin (POMC), and the interaction of α-MSH with its highly pleomorphic receptor MC1R, which mediates melanocyte functions including pigmentary phenotype and proliferation (82). p53 is believed to stimulate the POMC promoter in response to UV exposure, and mice genetically deficient in p53 fail to exhibit the normal tanning response. Accordingly, it is possible that p53 may function as, in addition to an inducer of apoptosis in UV-damaged cells, a sensor/effector of the tanning response, and even potentially as a trigger for certain pathologic forms of melanization.
Merkel Cells
Embryology
Although the origin of Merkel cells is unclear, it seems likely that they are differentiated from stem cells in the epidermis (98,99). The alternative hypothesis is that Merkel cells derive from the neural crest (98,100,101). The favored hypothesis, however, is that they arise between weeks 8 and 12 of gestational age from precursor stages of epithelial cells of early fetal epidermis that still express simple epithelial cytokeratins. In situ differentiation from epidermal ectoderm versus immigration of cells from the neural crest is supported by studies of fetal skin without Merkel cells transplanted to nude mice and subsequently found to contain mature Merkel cells within the engrafted tissue (102). Some Merkel cells detach from the epidermis and migrate temporarily into the upper dermis, where some of them associate with small nerves.
Normal Microanatomy
Merkel cells are present within the basal cell layer of the epidermis, oral mucosa, and the bulge region of hair follicles (103). They are quite scarce, irregularly distributed, and occasionally arranged in groups, often at tips of rete ridges (Fig. 3-18A), in eccrine glandular ridges of glabrous skin, in “Haarscheiben” of hairy skin, within beltlike clusters of hair follicles, and in certain mucosal tissues (104). It is assumed that the Merkel cell may represent a rudimentary touch receptor, more important in lower vertebrates (e.g., particularly in relationship to vibrissa hairs). The Merkel cell cannot be recognized in light microscopic sections; however, in silver-impregnated sections, the meniscoid neural terminal that covers the basal portion of each Merkel cell can be seen as a Merkel disk (105). A sensory nerve fiber may terminate at the disk. Immunohistochemistry for low-molecular-weight cytokeratins (CKs) such as CK8, -18, -19, and -20 more typical of simple epithelial cells than keratinocytes permits differential identification of Merkel cells in tissue sections (78). The most useful of these filamentous proteins is CK20, which has been shown to be a highly specific marker for Merkel cells because in human skin no other cell type expressing CK20 exists (103) (Fig. 3-18A).
Figure 3-18 A: Immunohistochemistry with cytokeratin 20 highlights Merkel cells in the glabrous epithelium of the digit. They are often distributed, forming small groups at the tip of the rete ridges. B: By electron microscopy, Merkel cells contain characteristic neurosecretory granules.
By electron microscopic examination, Merkel cells usually are located directly above the basement membrane (106). They possess small, electron-dense, membrane-bound neurosecretory-type granules, strands of perinuclear intermediate filaments, and occasional desmosomes on their cell membranes connecting them with neighboring keratinocytes (Fig. 3-18B). The electron-dense granules vary in size between 80 and 200 nm and tend to be located at the side of cytoplasm in contact with axon terminals. Intermediate-type junctions are noted between axon terminals and Merkel cells.
Regional Variation
Merkel cells are most heavily concentrated in skin with high hair density and in glabrous epithelium of the digits, lips, regions of the oral cavity, and the outer root sheath of the hair follicle. These cells are present in mammals as so-called “touch-domes,” also known as “Haarscheiben,” a form of type I mechanoreceptor that surrounds tylotrich (large touch-sensitive) hairs and the rete ridge collars about external root sheaths of hair follicles.
An increase in Merkel cells is seen after chronic sun exposure and has also been described in the epidermal basal layer adjacent to prurigo nodularis, actinic keratoses, and tumors such as basal cell carcinomas (104).
Specialized Structure and Function
Merkel cells are specialized epithelial cells that react with the intermediate filament cytokeratin and desmosomal proteins (107,108). Of note, Merkel cells also express neuroendocrine markers such as chromogranin A, the matrix substance of the dense-core granules, as well as synaptophysin, supporting the view that they are epithelial neuroendocrine cells and that they may possess a neurosecretory function (104,109). Merkel cells also contain neuron-specific enolase and neurofilaments in their cytoplasm (110,111). The characteristic presence of specific cytokeratins (CK20) and neuroendocrine markers in Merkel cells is relevant to the diagnosis of Merkel cell carcinoma (primary neuroendocrine tumor of the skin), perhaps the only practical reason for the diagnostician to know about this mysterious cell type.
In addition to the expression of neuroendocrine markers, a variety of neurosecretory substances, in particular neuropeptides, that are stored within the dense-core granules have been demonstrated in Merkel cells. These neuropeptides include vasoactive intestinal polypeptide, calcitonin gene-related peptide (CGRP), serotonin, and substance P (104). Although some of these neurosecretory substances work as neurotransmitters, others have been demonstrated to promote growth and differentiation of various cutaneous cell types (104). However, any functional significance to their presence in human Merkel cells is unproven.
Langerhans Cells
Embryology
Langerhans cells are bone marrow-derived, dendritic, antigen-presenting cells that appear in the epidermis by 7 weeks of gestation, as documented by their positive staining for adenosine triphosphatase (ATPase) (Fig. 3-19). Although all Langerhans cells at this stage are ATPase positive, they are negative for their more characteristic and specific cell surface glycoprotein CD1a. At a gestational stage of 60 days, Langerhans cells begin to express CD1a reactivity. By 80 to 90 days, the number of CD1a-positive cells increases abruptly, and after 90 days, the expression of CD1a is approximately equivalent to the number of ATPase-positive Langerhans cells (112) (Fig. 3-20A, B). S100 protein is not found in the Langerhans cells of fetal epidermis, but it can be demonstrated within 1 day after delivery (113). By electron microscopy, Langerhans cells can be identified by 10 to 11 weeks of gestation on the basis of the presence of Langerhans granules (Fig. 3-20C).
Figure 3-19 An epidermal sheet, removed and viewed en face, after adenosine triphosphatase enzyme histochemical staining. Note numerous, evenly distributed dendritic Langerhans cells.
Figure 3-20 A: Anatomy of a human Langerhans cell. B: Typical dendritic morphology seen with immunohistochemical stain for CD1a and immunofluorescence detection of CD1a glycoprotein by confocal scanning laser microscopy. C: Transmission electron micrograph of Langerhans cell (top) and characteristic cytoplasmic granules (Birbeck granules) (bottom).
Normal Microanatomy
Langerhans cells may be presumptively observed in histologic sections stained with hematoxylin–eosin as clear cells in the suprabasal epidermis. However, they cannot be reliably distinguished from occasional intraepidermal T lymphocytes and macrophages, and their dendritic cytoplasmic processes cannot be resolved. Because Langerhans cell detection is difficult in conventional sections, special stains are generally required for their detection and enumeration. Several enzyme histochemical stains have historically been used to identify Langerhans cells and to differentiate them from melanocytes. These include ATPase (Fig. 3-19) and aminopeptidase (114). Langerhans cells are also positive for HLA-DR antigen and S100 protein, although the former also reacts with cells forming the acrosyringium (115), and the latter is found in melanocytes (Fig. 3-14). Langerhans cells can be demonstrated more specifically with monoclonal antibody to the prothymocyte differentiation cell surface glycoprotein CD1a when the antibody is labeled with either peroxidase or fluorescein (116–118) (Fig. 3-20A, B).
By electron microscopic examination, Langerhans cells show a markedly folded nucleus and no tonofilaments or desmosomes. Melanosomes are only rarely found in them, and, if they are, they always are located within lysosomes, indicating that they have been phagocytized (119). Of great interest is the regular presence of an organelle called the Langerhans or Birbeck granule in the cytoplasm of Langerhans cells (Fig. 3-20C). The size of these granules varies considerably, from 100 nm to 1 μm (120). The granule has the three-dimensional shape of a disk and often shows a vesicle at one end and occasionally at both ends. A cross section of the disk portion has the appearance of a rod, and, if a vesicle is attached to the rod at one end, the Langerhans granule has the highly characteristic appearance of a tennis racquet. The rod-shaped cross section has a central lamella showing cross striation with a periodicity of 6 nm (120). Langerhans cell granules form as a consequence of endocytotic, clathrin-associated invaginations of the cell membrane of Langerhans cells. This was first recognized on the basis of the presence of intradermally injected peroxidase within the granules of Langerhans cells, even though the peroxidase molecule cannot cross the cell membrane (121). In addition, on incubating Langerhans cells with a gold-labeled antibody directed against the CD1a glycoprotein of the cell membrane of the Langerhans cell it was found that the labeled CD1a was internalized and appeared within Langerhans granules (122). The histiocytes present in the cutaneous and visceral lesions of histiocytosis X contain Langerhans granules. These granules are indistinguishable in their electron microscopic appearance from those seen in epidermal Langerhans cells (123,124). Cells that have the ultrastructural features of Langerhans cells but lack the Langerhans granule have been called indeterminate cells. They react specifically with the monoclonal antibody to CD1a (125,126).
Regional Variation
Langerhans cells are present in the epidermis in a concentration similar to that of melanocytes, between 460/mm2 and 1,000/mm2. In contrast to melanocytes, their number does not increase but instead may decrease with repeated exposure to ultraviolet light (114). CD1a-positive Langerhans cells have been found to be less plentiful in trunk skin than extremity skin (127). However, there appears to be considerable site variation among individuals, and the best comparison for determination of site-matched normal Langerhans cell numbers may be uninvolved skin directly adjacent to the lesion under study (127). Langerhans cells are present not only in the skin but also in the oral mucosa, the vagina, the lymph nodes, and the thymus; occasionally, they are seen in the dermis (128). Their numbers may vary significantly in pathologic processes, in part as a result of local accumulation or their tendency actually to migrate on stimulation (129).
Specialized Structure and Function
Langerhans cells originate in the bone marrow and are functionally and immunologically related to the monocyte–macrophage–histiocyte series (130). Langerhans cells constitute 2% to 4% of the total epidermal cell population, and they tend to be evenly distributed such that their dendrites extend toward the epidermal surface in a uniform manner (131). A few Langerhans cells are present in the dermis of normal skin. Langerhans cells express immune response-associated antigens class II (HLA-DR in humans, Ia in mice) (132,133), leukocyte common antigen (134) Fc and C3 receptors, CD1a antigen (117–119), CD1c (M241) antigen (135), a membrane-bound ATPase (131), S100 protein, and actin-like and vimentin filaments. These markers are compatible with an active role in cutaneous immunity.
Langerhans cells have antigen-processing and antigen-presenting capacity. The recognition of a soluble protein and haptenized antigens by T lymphocytes requires the initial uptake and processing by an HLA-DR–positive cell like the Langerhans cell, which then presents immunologically relevant signals to the T lymphocytes. This often involves active migration of the antigen-bearing Langerhans cell from the epidermis into the dermis, where transit to draining lymph nodes occurs via lymphatic channels. In the lymph node, naive T cells are plentiful for receipt of the antigenic signals communicated by the Langerhans cells in a class II (HLA-DR)–dependent manner. Such T-cell sensitization results in the formation of memory T cells with the ability to recall and respond to the specific antigenic peptide on reexposure or challenge. This mechanism is believed to provide an important component to normal cutaneous immunosurveillance, as well as the underpinning of the contact hypersensitivity reaction. However, recent studies suggest a more complex function for Langerhans cells in normal skin homeostasis, where they are involved in maintenance of tolerance through activation and proliferation of skin-resident regulatory T cells (136).
Ultraviolet radiation interferes with the antigen-presenting capacity of Langerhans cells. After UV irradiation fewer Langerhans cells could be demonstrated in the skin (137). It is likely that this initial decrease of Langerhans cells is due to a temporary loss of membrane markers rather than to a destruction of the cells. Chronic repeated exposure to UV light may result in actual depletion of cells, contributing to potential for carcinogenesis as a result of impaired immunosurveillance. Similar depletion of Langerhans cells may result from application of potent topical corticosteroids (138) and in the setting of acquired immunodeficiency syndrome (139). With respect to the latter, viral particles have been identified in Langerhans cells (140), which, it is interesting to note, express one of the HIV receptors, CD4 (141). Replenishment of Langerhans cells after depletion has been accomplished by application of topical retinoids (142).
Basement Membrane Zone
Normal Microanatomy, Ultrastructure, and Molecular Structure
The basement membrane is an inconspicuous structure of enormous functional significance, bonding together the protective epidermal layer and adnexal structures with the adjacent dermis. A subepidermal basement membrane zone not visible in sections stained with hematoxylin–eosin is seen on staining with the periodic acid Schiff (PAS) stain or by immunofluorescence as a 0.5- to 1.0-μm-thick homogeneous band (143) (Fig. 3-21). Its positive PAS reaction indicates a relatively large number of neutral mucopolysaccharides in this zone. Furthermore, impregnation with silver nitrate reveals a meshwork of reticulum fibers in the uppermost dermis. Staining with alcian blue, which identifies the band of polysaccharides and the reticulum meshwork, reveals that the band of polysaccharides is located above the reticulum layer (144). The light microscopic PAS-positive subepidermal basement membrane zone appears heterogeneous on electron microscopy (Fig. 3-22). The plasma membrane at the undersurface of basal cells shows hemidesmosomes (“half-desmosomes”) possessing only one intracytoplasmic attachment plaque, to which tonofilaments from the interior of the basal cell are attached (Fig. 3-22). The cytoskeletal intermediate filaments within basal keratinocytes that insert into hemidesmosomes are composed predominantly of keratins 14 and 5 (145,146) (Fig. 3-23). Hemidesmosomes contain plaque proteins involved in intermediate filament anchorage including bullous pemphigoid antigen 1 (BPAG1; 230 kD) and plectin, transmembranous proteins including bullous pemphigoid antigen 2 (BPAG2; 180 kD), and integrin α6β4. Additional components of the hemidesmosomes include p200, a noncollagenous N-glycosylated acidic protein that is distinct from subunits of type VI collagen (147), and other molecules not yet fully characterized. Bullous pemphigoid antigens are easily demonstrated by immunofluorescence as a smooth linear band that separates the epidermal and dermal layers (Fig. 3-21B). Beneath the plasma membrane of the basal cells, a rather electron-lucent zone called the lamina lucida separates the trilaminar plasma membrane, about 8 nm wide, from the more electron-dense component of the basement membrane, or lamina densa (143). Anchoring filaments extend from the basal cell plasma membrane to the lamina densa, traversing the lamina lucida (Fig. 3-23). Anchoring filament components include BPAG2 and α6β4 integrin, which extend from the basal cell membrane into the lamina lucida. BPAG2 exhibits features of a collagen molecule, and it is also referred to as collagen XVII. The 97-kD/120-kD protein ladinin, an important antigen in linear immunoglobulin A (IgA) dermatosis (lamina lucida type), is considered to represent a degradation product of the 180-kD bullous pemphigoid antigen (BPAG2) (148). Other proteins in the lamina lucida that are associated with these anchoring filaments include laminin 5 (also called kalinin, nicein, or epiligrin), uncein (defects in uncein are known to be involved in the recessive junctional type of epidermolysis bullosa), laminin 1, and nidogen (entactin). Although the molecular binding interactions are undoubtedly complex, it is known that α6β4 integrin is a receptor for laminin 1 and 5, and that laminin 5 (epiligrin) is a ligand for α3β1 integrin, which is also expressed by basal keratinocytes. Such adhesive interactions are likely to contribute to the anchoring of basal cells to underlying lamina lucida. Nidogen within the lamina lucida is a 150-kD protein that facilitates adhesion between specific domains of laminin and type IV collagen within the adjacent lamina densa. Anchoring fibrils are short, curved structures with an irregularly spaced cross-banding of their central portions (149). They fan out at either end, the distal part inserting into the lamina densa and the proximal part terminating in the papillary dermis or looping around and merging in the lamina densa. They insert into amorphous patches containing type IV collagen, which is the main component of the lamina densa (149). The lamina densa itself is anchored to the underlying dermis in part by anchoring fibrils. The anchoring fibrils derive, at least in part, from the dermis and contain type VII collagen (150). The anchoring fibrils connect with islet-like anchoring plaques in the subjacent dermis. These anchoring plaques have a basement membrane-like composition (146). These anchoring structures are interwoven with the dermal interstitial type I and type III collagen fibers, resulting in the adhesion of the basement membrane to the dermis.
Figure 3-21 A: Demonstration of normal basement membrane zone separating epidermal and dermal layers by periodic acid Schiff stain. B: By direct immunofluorescence staining for the basement membrane–associated structural protein bullous pemphigoid antigen, a linear band is observed along the dermal–epidermal junction.
Figure 3-22 Transmission electron microscopy of the basement membrane separating the epidermis and dermis. Hemidesmosomes, lamina lucida, lamina densa, and anchoring fibrils can all be seen in this image.
Figure 3-23 Diagram illustrating the different components of the desmosome and basement membrane zone. DP, desmoplakin; DSC, desmocollin; DSG, desmoglein; PG, plakoglobin; PP, plakophilin; DSC-1, desmocollin-1; DSG-1, desmoglein-1; DSG-3, desmoglein-3; BP, bullous pemphigus; PG, pemphigoid gestationis; CP, cicatrical pemphigus; LPP, lichen planus pemphigoides; EBS, epidermolysis bullosa simplex; EB, epidermolysis bullosa; EBA, epidermolysis bullosa acquisita; SSSS, staphylococcal scalded skin syndrome.
In addition to anchoring fibrils, elastic fibers consisting of microfibril bundles and individual microfibrils approximately 10 nm in diameter are attached to the undersurface of the lamina densa. Three varieties of elastic tissue exist: oxytalan, elaunin, and elastic fibers (151). The oxytalan fibers consisting of microfibrils form a thin, superficial network perpendicular to the dermoepidermal junction. They originate from a plexus of elaunin fibers located parallel to the dermoepidermal junction in the upper dermis. The elaunin fibers are connected with the thicker elastic fibers of the middle and deep dermis. Effective anchoring of the epidermis to the dermis is a function largely of the anchoring fibrils; anchoring of the basement membrane by oxytalan fibers is quite sparse (152). Defects in basement membrane zone adhesive molecules due to autoantibodies or gene defects may result in disorders characterized by dermal–epidermal separation, as in bullous pemphigoid (BPAG1 and 2 = targets), cicatricial pemphigoid and Herlitz-type junctional epidermolysis bullosa (laminin 5 and uncein = target), and epidermolysis bullosa acquisita, dystrophic epidermolysis bullosa, bullous systemic lupus erythematosus, and some cases of linear IgA disease (type VII collagen = target). There are likely to be numerous other antigenic targets in the basement membrane zone that are relevant to human disease, and our understanding of these new molecules is actively evolving. For example, the 200 kDa protein p200 has recently been implicated in a disorder initially named anti-p200 pemphigoid. This autoimmune subepidermal blistering disease was originally thought to target p200 (153,154), although recently, the C-terminus of laminin γ1 has been identified as target antigen in anti-p200 pemphigoid and the disease was renamed as anti-laminin γ1 pemphigoid (155). The immunobullous disorders with their respective targets are summarized in Fig. 3-23, and discussed further in Chapters 6 and 9.
In addition to their critical adhesive junctions, basement membrane related proteins have recently been shown to subserve other important functions. Certain protein components of the hemidesmosomes have more than just a structural role; for example, integrin α6β4 is able to transduce signals from the extracellular matrix to the interior of the basal cells modulating the organization of their cytoskeleton and their proliferation, apoptosis, and differentiation (156).
Hair Follicles
Embryology
Hair germs, or primary epithelial germs, are first observed in embryos in the eyebrow region and the scalp during the third month of gestation (157). The general development of hair begins in the fourth fetal month in the face and scalp and gradually extends in a cephalocaudal direction. Thus, during the fourth month, while some hair follicles on the head are already well matured and are producing hair, most of those on the trunk are barely differentiated (158). In addition, new primary epithelial germs continue to form adjacent to more-established ones so that, in any section obtained from the beginning of the fifth month up to birth, hair structures in different stages of development are found (159).
Hair germs, or primary epithelial germs, in their earliest stage of development consist of a cluster of tightly aggregated, deeply basophilic cells in the basal cell layer of the epidermis. Subsequently, these develop into buds that protrude into the dermis (Figs. 3-24 and 3-25). Beneath each bud lies a zone of plump mesenchymal cells from which the dermal hair papilla is later formed. As the primary epithelial germ grows deeper into the dermis under the inductive influence of the underlying mesenchymal cells, it forms first the hair peg and then, as the hair matrix cells and the dermal hair papilla develop, the bulbous hair peg (160). Ultrastructural observations suggest that the “hair germ mesenchymal cells” pull down the hair germ as they move deeper in the dermal mesenchyme (157).
Figure 3-24 Schematic overview of embryonic trichogenesis in human skin. (Courtesy of Dr. Michael Ioffreda.)
Figure 3-25 Histology of early trichogenesis, showing the formation of a primary epithelial germ with associated underlying mesenchymal condensation (1-μm-thick, plastic-embedded section).
As the bulbous peg stage is reached, differentiation occurs in the lower and upper portions of the hair follicle and in the overlying epidermis. Differentiation in the lower portion of the follicle leads to the formation of the hair cone and subsequently to the formation of the hair, the cuticle, and the two inner root sheaths. The hair canal in the upper portion of the hair follicle, located at the level of the upper dermis, is formed by the premature death of the central core cells before they have become keratinized. In contrast, the intraepidermal portion of the hair canal is produced by means of premature keratinization and subsequent dissolution of the matrix cells of the cordlike hair canal extending obliquely through the epidermis. By the time the hair cone has reached the upper portion of the hair follicle, the hair canal is already open within the dermis and epidermis (161).
The hair follicles grow at a slant and, in the late hair peg or early bulbous stage, develop two or three bulges on their undersurface. The lowest of the three bulges develops into the attachment for the arrector pili muscle and is associated with the follicular stem cell niche; the middle bulge differentiates into the sebaceous gland. The uppermost bulge, if present, either involutes or develops into an apocrine gland. Apocrine glands develop only in certain regions (162). The intraepidermal portion of the hair canal seems to form by lysosomal digestion of cellular cytoplasm analogous to the formation of the intraepidermal eccrine duct and of the intrafollicular apocrine duct (161).
In sections treated with the dopa reaction or stained with ammoniated silver nitrate, melanocytes are distributed at random in primary epithelial germs and in hair pegs. During the bulbous peg stage, the melanocytes concentrate in the so-called pigment matrix region (the basal cell layer lying on top of the dermal hair papilla) and to a lesser degree in the lower hair bulb located lateral to the dermal hair papilla (159).
Normal Microanatomy
General Anatomic Features
The hair follicle, with its hair in longitudinal sections, consists of three parts: the lower portion, extending from the base of the follicle to the insertion of the arrector pili muscle; the middle portion, or isthmus, a rather short section, extending from the insertion of the arrector pili to the entrance of the sebaceous duct; and the upper portion, or infundibulum, extending from the entrance of the sebaceous duct to the follicular orifice (Figs. 3-26 to 3-33). The lower portion of the hair follicle is composed of five major portions: the dermal hair papilla; the hair matrix; the hair, consisting inward to outward of medulla, cortex, and hair cuticle; the inner root sheath, consisting inward to outward of inner root sheath cuticle, Huxley layer, and Henle layer; and the outer root sheath (Figs. 3-27 and 3-28). This bulb region gives rise to the formation of the hair shaft that occupies the overlying follicular canal (Figs. 3-27 and 3-28). The hair shaft is predominantly composed of keratin. The large keratin multigene family comprises the epithelial keratins and the hair keratins, the latter involved in the formation of hard keratinized structures such as hair and nail. The human hair keratin family consists of nine type I and six type II members, whose genes are organized as distinct clusters within the type I and type II epithelial keratin gene domains (163,164).
Figure 3-26 Low-power view of a hair follicle to illustrate its four zones. From bottom to top: the hair bulb, the suprabulbar zone (at which point the various layers of the follicle start to differentiate), the isthmus (located between the arrector pili muscle and entrance of the sebaceous duct), and the infundibulum (the uppermost zone of the follicle, whose inferior boundary is represented by the entrance of the sebaceous duct).
Figure 3-27 Histology of the lower portion of the human hair follicle. A: The terminal anagen hair bulb is usually located in the deep dermis or superficial subcutaneous tissue. B: The basophilic hair matrix surrounds the dermal papillae. C: Note the pigmented and dendritic melanocytes in the suprapapillary matrix. The typically mucinous inferior portion of the follicular papilla (stalk) is continuous with the fibrous root sheath.
Figure 3-28 A: Suprabulbar portion (lower segment) of the hair follicle. The various layers of the anagen hair can be recognized. From the inside to the periphery: the medulla (M) when present, the cortex (C), the cuticle (Cu) that includes the hair shaft cuticle and the cuticle of the inner root sheath (IRS), the Huxley and Henley layers of the IRS, the highly glycogenated outer root sheath (ORS), and the glassy vitreous layer (VL). B: Note the loss of nuclei in the hair shaft and inner root sheath.
Figure 3-29 Isthmus of a hair follicle. Its upper limit is marked by the sebaceous gland opening. In the mid portion of the isthmus, the abrupt desquamation of the inner root sheath (gray blue) takes place. Above it, the outer root sheath cornifies (dark pink) without formation of a granular layer (trichilemmal keratinization).
Figure 3-30 A: Sebaceous gland and sebaceous duct opening into a hair follicle. B: Transmission electron microscopy of a sebaceous lobule. Lipid is visible as rounded, homogeneous bodies filling the cytoplasm of mature sebocytes.
Figure 3-31 Vellus hair. The bulb of these small hair follicles is located in the upper dermis. As terminal hairs, vellus hairs go through the entire hair cycle.
Figure 3-32 Diagram of the hair cycle. From left to right: anagen hair, catagen hair, and telogen hair.
Figure 3-33 Section of a catagen/telogen hair. Note the club-shaped cornifying hair shaft with serrated borders. There are numerous apoptotic nuclei in the hair epithelium.
Cycles of Hair Growth
The size of the hair follicles varies from long and thick terminal hairs with their bulbs in the fat to thin, short, and often hypopigmented vellus hairs with bulbs located in the upper dermis (Fig. 3-31). Regardless of size, every follicle goes through the three phases of the “hair cycle.” The histologic appearance of the hair follicle changes considerably during the hair cycle, which causes the hair to turn from an anagen hair into a catagen hair, then into a telogen hair, and finally into a new anagen hair (Chapter 18). In the adult scalp, the anagen stage—the phase of active growth—lasts at least 3 years; catagen—the phase of regression—lasts about 3 weeks; and telogen—the resting period—lasts about 3 months. At any one time, approximately 84% of scalp hairs are in anagen stage, 2% in catagen, and 14% in telogen (165). These ratios are important in evaluating abnormalities in the hair cycle in biopsies of partially alopecic scalp. The daily hair growth rate averages about 0.4 mm on the scalp.
During its active growth, or anagen, stage the hair follicle shows at its lower pole a knoblike expansion, the hair bulb, composed of matrix cells and melanocytes. A small, egg-shaped dermal structure, the dermal hair papilla, protrudes into the hair bulb (Fig. 3-27). The papilla induces and maintains the growth of the hair follicle (166). Because of the presence of large amounts of acid mucopolysaccharides in its ground substance, the dermal hair papilla stains positively with alcian blue and metachromatically with toluidine blue. Because positive staining with alcian blue takes place at pH 2.5 and 0.5, it can be concluded that the ground substance of the hair papilla contains nonsulfated acid mucopolysaccharides, such as hyaluronic acid, and sulfated acid mucopolysaccharides, such as chondroitin sulfate (167,168). In addition, there is considerable alkaline phosphatase activity in the hair papilla during the anagen stage as a result of the presence of large numbers of capillary loops (168,169). In persons with dark hair, large amounts of melanin can be seen in the dermal hair papilla situated within melanophages.
Bulb and Lowermost Portion of the Hair Follicle
The pluripotential cells of the hair matrix present in the hair bulb give rise to the hair and to the inner root sheath. In contrast, the outer root sheath represents a downward extension of the epidermis. The cells of the hair matrix have large vesicular nuclei and a deeply basophilic cytoplasm. Dopa-positive melanocytes are interspersed mainly between the basal cells of the hair matrix lying on top of the dermal hair papilla and, to a lesser degree, between the basal cells of the hair matrix located lateral to the hair papilla (159). Melanin, varying in quantity in accordance with the color of the hair, is produced in these melanocytes and is incorporated into the future cells of the hair through phagocytosis of the distal portion of dendritic processes by future hair cells. This transfer of melanin is analogous to that observed from epidermal melanocytes to keratinocytes.
As they move upward, the cells arising from the hair matrix differentiate into six different types of cells, each of which keratinizes at a different level. The outermost layer of the inner root sheath, the Henle layer, keratinizes first, thus establishing a firm coat around the soft central parts. The two apposed cuticles covering the inside of the inner root sheath and the outside of the hair keratinize next, followed by the Huxley layer. The hair cortex then follows, and the medulla is last (170).
The hair medulla of human hair is often difficult to find by routine light microscopy because it may be discontinuous or even absent. It is more readily recognizable by polariscopic examination because, unlike the cortex, the only partially keratinized medulla contains hardly any doubly refractile structures (171). If the medulla is seen by light microscopy in human hairs, it appears amorphous because of only partial keratinization.
The hair cortex consists of cells that, during their upward growth from the hair matrix, keratinize gradually by losing their nuclei and become filled with keratin fibrils. The process of keratinization takes place without the formation of keratohyaline granules, as seen in the keratinizing epidermis, or of trichohyaline granules, as seen in the inner root sheath. Thus, the keratin of the hair cortex represents hard keratin, in contrast to the keratin of the inner root sheath, which, like that of the epidermis, represents soft keratin (172).
The hair cuticle (Fig. 3-28), located peripheral to the hair cortex, consists of overlapping cells arranged like shingles and pointing upward with their peripheral portion. The cells of the hair cuticle are tightly interlocked with the cells of the inner root sheath cuticle, resulting in the firm attachment of the hair to its inner root sheath. The hair and the inner root sheath move upward in unison (173).
The inner root sheath is composed of three concentric layers; from the inside to the outside, these are the inner root sheath cuticle, the Huxley layer, and the Henle layer (Fig. 3-28). None of these three layers contains melanin. All three layers keratinize, unlike the cells of the hair cortex and of the hair cuticle, by means of trichohyaline granules. These granules resemble the keratohyaline granules of the epidermis, although they stain eosinophilic, in contrast to the basophilic-staining keratohyaline granules of the epidermis. Similar granules may be noted in the pathologic process of epidermolytic hyperkeratosis. Closest to the hair is the single-layered inner root sheath cuticle, consisting of flattened overlapping cells that point downward in the direction of the hair bulb. Because the cells of the hair cuticle point upward, these two types of cells interlock tightly. Trichohyaline granules are few in the inner root sheath cuticle cells. The Huxley layer, which usually consists of two rows of cells, develops numerous trichohyaline granules at the level of the keratogenous zone of the hair. The Henle layer, which is only one cell layer thick and the first layer to undergo keratinization, already has many trichohyaline granules at its emergence from the hair matrix (174) (Fig. 3-27). After having become fully keratinized, the cells of all three layers composing the inner root sheath disintegrate when they reach the isthmus of the hair follicle, which extends from the area of attachment of the arrector pili muscle to the entrance of the sebaceous duct. The cells of the inner root sheath thus do not contribute to the emerging hair (175).
The outer root sheath extends upward from the matrix cells at the lower end of the hair bulb to the entrance of the sebaceous duct, where it changes into surface epidermis, which lines the upper portion, or infundibulum, of the hair follicle. The outer root sheath is thinnest at the level of the hair bulb, gradually increases in thickness, and is thickest in the middle portion of the hair follicle, the isthmus. In its lower portion, below the isthmus, the outer root sheath is covered by the inner root sheath and does not undergo keratinization. The outer root sheath cells have a clear, vacuolated cytoplasm because of the presence of considerable amounts of glycogen (Fig. 3-28). In contrast to the surface epidermis lining the infundibulum, which contains active, melanin-producing melanocytes in its basal layer, the basal layer of the outer root sheath contains only inactive, amelanotic melanocytes demonstrable with toluidine blue. However, these inactive melanocytes can become melanin-producing cells after skin injuries, such as dermabrasion, when they increase in number and migrate upward into the regenerating upper portion of the outer root sheath and into the regenerating epidermis.
Follicular Isthmus and Infundibulum
In the middle portion of the hair follicle, the so-called isthmus, which extends upward from the attachment of the arrector pili muscle (so-called bulge region) (Fig. 3-29) to the entrance of the sebaceous duct, the outer root sheath is no longer covered by the inner root sheath, which by then has keratinized and disintegrated. The outer root sheath therefore undergoes keratinization. This type of keratinization, referred to as trichilemmal keratinization, produces large, homogeneous keratinized cells without the formation of keratohyaline granules (Fig. 3-29). Trichilemmal keratinization is found also in catagen and telogen hairs, and in trichilemmal cysts and trichilemmal tumors. The bulge region at the site of the arrector pili muscle insertion is poorly defined in human follicles and difficult to detect in routine sections. When it is visualized, it consists of several knobby protuberances and ridges composed of relatively undifferentiated follicular keratinocytes with putative stem cell capability. Stem cells in the bulge region participate in the regeneration of the hair follicle during cycling. The cells reside in a niche that includes the dermal papilla and dermal sheath. Interactions between hair follicle epithelial and dermal cells are necessary for hair follicle morphogenesis during development and in the hair cycle (176).
The upper portion of the hair follicle above the entrance of the sebaceous duct, the infundibulum, is lined by surface epidermis, which, like the sebaceous duct, undergoes keratinization with the formation of keratohyaline granules. The infundibulum contains a number of dendritic nonkeratinocytes on ultrastructural examination, and immunohistochemistry reveals numerous CD1a-positive Langerhans cells within the infundibula of normal adult anagen hairs.
The glassy or vitreous layer forms a homogeneous, eosinophilic zone peripheral to the outer root sheath (Fig. 3-28). Like the subepidermal basement membrane zone, it is PAS positive and diastase resistant, but it differs from the subepidermal basement membrane zone by being thicker and visible with routine stains. It is thickest around the lower one-third of the hair follicle. Peripheral to the vitreous layer lies the fibrous root sheath, which is composed of thick collagen bundles.
Sebaceous Glands
The sebaceous glands are well developed at the time of birth, most likely because of maternal hormones. After a few months, they undergo considerable involution. At puberty, as a result of increased androgen output, the sebaceous glands become greatly enlarged (177). This normal cycle may also occur in certain pathologic conditions, such as nevus sebaceus.
A sebaceous gland may consist of only one lobule but often has several lobules leading into a common excretory duct composed of stratified squamous epithelium (Fig. 3-30A). Sebaceous glands, being holocrine glands, form their secretion by decomposition of their cells. In sebaceous glands of the skin, the pilosebaceous follicle into which the sebaceous duct leads may possess a large hair or a vellus hair that may be too small to reach the skin surface. There is no relationship between the size of the sebaceous gland and the size of the associated hair. For example, in the center of the face and on the forehead, where the sebaceous glands are very large, the associated hairs are of the vellus type. Each sebaceous lobule possesses a peripheral layer of cuboidal, deeply basophilic cells that usually contain no lipid droplets (Fig. 3-30B). The more centrally located cells contain lipid droplets, which can be detected if lipid stains are used on formalin-fixed frozen sections, but in routinely processed sections in which the lipid has been extracted, the cytoplasm of these cells appears as a delicate network of fine cytoplasmic vacuoles. The nucleus is centrally located. In the portion of the lobule located closest to the duct, the cells disintegrate.
The composition of lipids in the sebaceous glands is not uniform. Thus, under polarized light, doubly refractile lipids may be present in small to moderate amounts or may be absent (178). Histochemical examination reveals the presence of triglycerides and small amounts of phospholipids. Esterified cholesterol is present, but there is no free cholesterol. Waxes are also present, but they are not identifiable by histochemical means (178). Evidence of active lipid synthesis and storage may be observed by electron microscopy (179,180).
Lysosomal enzymes bring about the physiologic autolysis that occurs in the holocrine secretion. Histochemical staining of electron microscopic sections for lysosomal enzymes, such as acid phosphatase and aryl sulfatase, reveals an increasing number of lysosomes as the sebaceous cells become more lipidized. In the disintegrating cells located in the preductal region, the acid phosphatase and aryl sulfatase activity is most pronounced, but this activity is largely outside of lysosomes because the lysosomes have released their contents in their function as “suicide bags” (181). Prior to the sudden disintegration of the sebaceous cells an abrupt conversion of –SH to S–S linkages of proteins occurs in sebaceous glands just as in epidermis and hair (182).
Regional Variation
Hair follicles vary considerably in different anatomic regions and according to age and sex. In adults, deep anagen hairs (extending into subcutaneous fat) are typically found on the scalp (see Fig. 3-59) and male beard area, whereas vellus hairs typify the female face and nonbeard-area facial regions of men. Hair of extremities and trunk generally can be differentiated from scalp by the more superficial location of the bulbs and diminished density. Sebaceous glands are present everywhere on the skin except on the palms and soles. On the skin, they are found in association with hair structures. In addition, free sebaceous glands that are not associated with hair structures occur in some areas of modified skin, such as the nipple and areola of both the male and female breasts. There, they occur over the entire surface of the nipple and in Montgomery areolar tubercles, each of which contains several sebaceous lobules in association with a lactiferous duct. Free sebaceous glands also may be found on the labia minora and the inner aspect of the prepuce. Modified sebaceous glands called Tyson glands have been reported to occur on the coronal sulcus of the penis (183). The not infrequent presence of free sebaceous glands on the vermilion border of the lips and on the buccal mucosa is known as Fordyce condition. The meibomian glands of the eyelids are modified sebaceous glands (see Fig. 3-62A).
Specialized Structure and Function
Hair Cycle
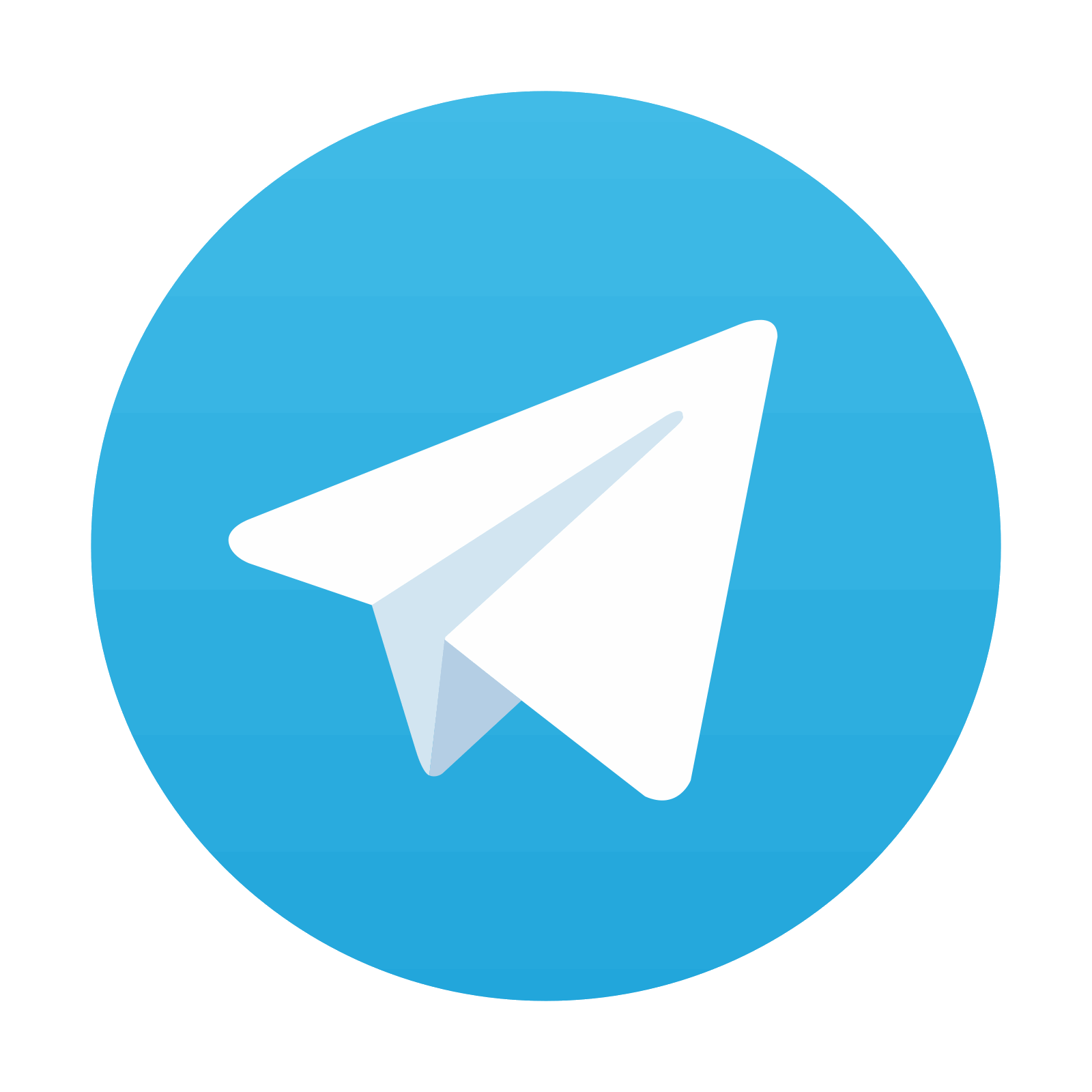
Stay updated, free articles. Join our Telegram channel
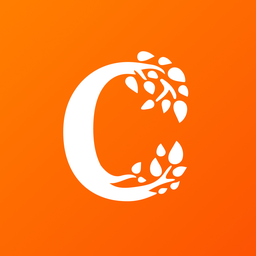
Full access? Get Clinical Tree
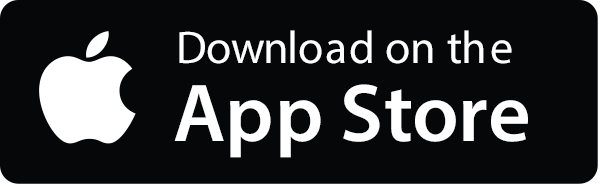
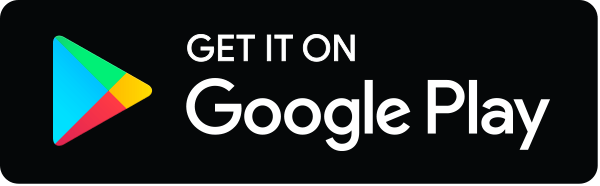