Desired feature of delivery system
Description of feature
Safety
Achieving desired effect with minimal morbidity
Practicality (ease)
Readily adoptable by broad range of users, while also maintaining patient safety
Minimal invasiveness
Limited procedural trauma may be more readily translated into patients with advanced disease, e.g., heart failure
Achieves delivery at a critical concentration
Allows delivery of a biological agent at a threshold level to ensure effect
Appropriate regional distribution
Dependent on clinical need, provides either regional or global tissue/organ delivery
Homogeneity of expression and biologic effect
Ensures that all cells within an targeted area are impacted (rather than patchy distribution)
Limited systemic exposure and/or toxicity
Minimizes induction of systemic responses (e.g., immunologic) or off-target consequences of accumulation in nontarget tissue
Cost effectiveness
Determined by technical and equipment aspects
Procedural repeatability
Determined by technical aspects such as invasiveness and cost in addition to biologic responses that limit the effect of repeat exposure
Once the critical features needed for an application have been determined, a type or class of vector can be selected. Until recently, gene therapy delivery agents were classified into two basic categories which were defined as (1) viral agent vectors and (2) nonviral vectors. Those gene transfer means which operated within viral delivery constructs employed the use of adenoviruses, oncoretroviruses, lentiviruses, and adeno-associated viruses or AAVs [4, 6]. Although evolutionarily adept at the delivery of genetic material into both eukaryotic and prokaryotic cells, viruses as bioengineered vectors for the transfer of therapeutic deoxyribonucleic acid (DNA) sequences are not without significant concerns, which has directed the field toward alternative vectors ranging from naked DNA sequences to liposomal delivery structures and even pretransplantation infusion of allogenic donor cells termed donor-specific transfusion (DST) which are thought to induce anergy and T cell clonal deletion .
Naked DNA
Perhaps the simplest method of gene transfer which does not require a viral vector or additional conjugate carrier is a form of so called “naked” transfection. Here, the DNA itself is both the transport element and coding sequence which is delivered to target cells often through a more stabilized circular plasmid structure (pDNA), which prevents rapid degradation of the DNA once within the cell. An array of techniques have been developed in order to optimize the transfection of naked DNA into cells, all of which have shown relatively limited efficacy when it comes to in vivo application.
The common theme of delivery involves the transient induction of increased cell membrane permeability through the application of either physical stress or simple chemical compounds on cells which challenge the cell membrane integrity for short periods of time. Basic physical or nonchemical methods encompass electroporation, micro-fluid platforms cell squeezing, and sonoporation. New advances in electroporation as well as gene administration techniques have increased naked DNA transfection efficiencies [4, 8].
Intravascular delivery of DNA into mice and nonhuman primates has been demonstrated to result in increased transgene delivery to kidney and muscle cells of up to 50 % [4, 9]. The use of electroporation produces a tenfold increase in intramuscular DNA delivery gene expression. However, this enhanced delivery is accompanied by cell death, as evidenced by increases in serum creatinine kinase levels [6, 10].
One of the major drawbacks of naked DNA therapy is the transient nature of expression that lasts approximately no longer than 2–10 days [4, 6, 9]. Increasing injection volume with rapid delivery can prolong gene expression up to 12 weeks. However, the volume requirement of 100 ml/kg body weight delivered over 15 s makes the administration method difficult for clinical implementation [4, 6, 11]. The induction of cell damage with current delivery techniques makes naked DNA gene therapy use problematic in transplantation. The increased cell death can lead to activation of the immune response, prompting allograft rejection and causing significant organ dysfunction. Improvement in naked DNA delivery system makes this a possible option viable for use in solid organ transplantation and VCA.
Cationic Polymer Vectors
An alternative to the transfection of naked DNA, which can augment the efficacy of delivery, is through the application of carrier which is complexed with the DNA sequence that can increase the uptake by target cells.
At this time, in order to gain sufficient and reproducible delivery of pDNA into the nucleus of the desired human cells both in a safe and an efficient manner, pDNA requires a carrier. A spectrum of chemically synthesized cationic polymer vectors has been developed by different groups which have been assayed to determine if certain complexes possess an advantage over another and if any can compete with the more effective viral-directed gene transfer [12]. Cationic polymer vectors or, often termed, polyplexes (polycation/DNA complexes) are formed between cationic polymers and DNA through electrostatic interactions, which physically compact the DNA into a smaller structure. These types of compacted poly-charged structures are by far the most widely used nonviral gene delivery vector system [13].
A myriad of complex variables affect gene transfection efficiency when using cationic polymer vectors such as molecular weight, surface charge, charge density, hydrophilicity of the complex, structure of cationic polymers/polyplex, and those specific features which those cells or tissues being target for gene therapy possess [4, 6, 13]. Understanding the dynamic features of cationic polymers and their interaction with both coding DNA and target cellular elements requires optimization of each polyplex for each target system in order to optimize gene transfection efficiency for that specific assay. Currently, there are several cationic polymeric vectors most commonly used in model systems gene transfer. Among these cationic polymeric vectors are:
1.
Polyethylenimine (PEI): A cationic polymer vector that maintains a repeating subunit which contains both an NH2 amine group separated by two carbon aliphatic CH2CH2 spacers. Often utilized within liquid adhesives detergents, bonding agents, and cosmetics, PEI’s positive charged polymer properties allows it to electrostatically interact with the net negative charge of cell membranes. This interaction permits PEI-coated materials to bind cells weakly for attachment. Additionally, this attraction allows PEI complexed with DNA to interact with the anionic cell membrane, allowing for endocytosis of the polyplex. Following endocytosis, the polyplex carrier within the endocytic vesicle utilizes NH2 amine groups as sites of protonation. This alteration in pH of the vesicle offsets osmotic equilibrium by altering the flux of ions, and subsequently, the hypoosmotic vesicles become swollen with an influx of equilibrating water influx, leading to rupture. The DNA is then released and can migrate into the nucleus [13]. Although capable of simple delivery of a DNA polyplex into cells, the constructs through its mechanism of delivery are cytotoxic at both the cell and mitochondrial membrane levels among a spectrum of mammalian cells at given carrier concentrations [14].
2.
Poly-(l-Lysine) (PLL): Similar to PEI, this polycationic vector has demonstrated a capacity to induce apoptosis in a range of human cell lines (Jurkat T cells; epithelial cells transformed with SV40 large T antigen (THLE3-hepatocytes); human umbilical vein endothelial cell, HUVEC). However, the mechanism of apoptosis differs in PLL-treated cells when PEI to those which received PEI. PLL is thought to induce apoptosis through cytochrome C accumulation as well as subsequent activation of protein kinases within recipient cells [15, 16].
3.
Chitosan: A polycationic polymeric structure isolated from specific sugars within crustaceans skeletons. Following Na(OH) processing, the linearly oriented polysaccharides is composed of glucosamine and N-acetyl-glucosamine units, which has allowed biomedical companies to utilize this substrate in wound care and hemostasis agents. For example, forms of chitosan have been approved as a wound dressing (Tegasorb® by 3M) and hemostatic patch (Hem-con® by HemCon) as well as within other fields of skin, nerve, cartilage, and bone regeneration [17]. Current application and research have not only shown that the use of chitosan nanoparticles greatly increases the transport of drugs through tissues but that this polysaccharide is also able to coat DNA for cellular delivery. Attractive qualities associated with chitosan-derived vectors pivot around the fact that these complexes are naturally derived, biocompatible, biodegradable, mucoadhesive, and nontoxic in polymer form [5].
4.
Polyamidoamine (PAMAM): First described in 1985 as “A New Class of Polymers: Starburst-Dendritic Macromolecules,” they are now the most common class of dendrimers in biotechnology applications and materials science engineering [18]. Structurally consisting of a varying alkyl-diamine core and branching side chains containing tertiary amines, these dendrimers are thought to result in higher gene transfection efficiency and lower cytotoxicity compared with other cationic polymers [19]. PAMAM, initially thought to have too complicated synthesis technology to be clinically useful, now has a more promising future with the advent of hyperbranched polyamidoamine (h-PAMAM). This form is synthesized by a simpler one-pot method yet has similar gene delivery properties with PAMAM. In addition, through a polyethylene glycol (PEG)ylation modification, the previously described cytotoxicity has been reduced [20].
At the present time, polycationic vectors are not as efficient as viral vector delivery systems within gene transfer modeling [13]. Researchers in the field have seen an increase in transfection efficiency with PEGylated polycationic polymeric forms which allows an increased total quantity of amount of pDNA uptake by target cells. A wide range of polymeric vectors have been utilized to deliver therapeutic genes in vivo. The modification of polymeric vectors has also shown successful improvements in achieving target-specific delivery and in promoting intracellular gene transfer efficiency. Various systemic and cellular barriers, including serum proteins in blood stream, cell membrane, endosomal compartment, and nuclear membrane, were successfully circumvented by designing polymer carriers having a smart molecular structure [15]. Cationic polymers display less toxicity associated with cytokine induction compared to their cationic lipid counterparts, which often elicit form of innate immune response through fatty acid inflammatory reactivity [4, 5].
Lipid Carriers
Another form of nonviral delivery vectors utilized in gene transfer was described in a 1963 publication of Nature as a negatively charged self-forming lipid membrane; this form of carrier has developed a spectrum of lipid-derived vectors such as liposomes, micelles, and nanoemulsions. Alterations in organic synthesis have allowed biochemists to manipulate these lipid elements to become net positive or cationic at the head region of the lipid, which allows for interaction with both the negatively charged DNA backbone and target cell membranes. As early as the 1980s, researchers were describing these synthetic cationic lipids such as N-[1-(2,3-dioleyloxy)propyl]-N, N,N-trimethylammonium chloride (DOTMA). At those times, studies described small unilamellar liposomes containing DOTMA which were capable of spontaneous interaction and incorporation with DNA. This interaction appeared to result in a lipid–DNA complex that was capable of 100 % DNA entrapment. Furthermore, DOTMA-enriched liposome/DNA complex could facilitate the fusion of the complex with the plasma membrane of cells, resulting in both uptake and expression of the DNA [21].
And since the discovery that phospholipids could form lamellar bilayer structures in aqueous systems, liposomes have become a prominent topic of research in gene delivery. The structure of these cationic lipid vectors defines their simplicity, versatility, and biocompatibility. Many fields, particularly medicine, have yielded successful breakthroughs through liposome delivery mechanisms with over 12 liposomal-based drug therapies on the market and over 20 in clinical trials worldwide that the vector has proven adequate safety and efficacy within human drug therapy [22]. From a production standpoint, the relatively simple preparation and various structural aspects of the liposome and lipid carriers have given rise to a reproduce mode of the internalization of a wide variety of biomolecules such as drugs, DNA, RNA, and even imaging probes [23].
Drawbacks concerning liposomal-mediated gene transfer have been published citing liposomes as having the capability of eliciting an immune response within the recipient tissues systems due to the expression of cytosine poly-guanine (CpG) bacterial motifs [24]. Moreover, in some cell-based and tissue-based systems, studies have described the necessity of delivering relatively high liposome construct concentrations in order to facilitate an adequate cellular transfection, resulting in measurable transgene expression. Here, limited transduction efficiency coupled with a reactive immune response to potentially inflammatory components of lipid-conjugated molecules suggests prospective concerns for irreversible cellular toxicity and subsequent systemic damage. Researchers investigating gene transfer in pancreatic islets cells, through liposomal–DNA complex vector delivery, described a concentration phenomenon which is both clinically relevant and concerning to gene modification allotransplantation efforts. This phenomenon describes an association of where the concentration of liposomal cations vectors necessary to achieve effective gene transfer directly results in the death of 50 % of islet cells receiving the therapy, in addition to impaired in vitro insulin release of the surviving transduced islets [4].
As the field continues to evolve further away from conventional vesicles toward yet another new generation liposomes, such as cationic liposomes, temperature-sensitive liposomes, and virosomes, researchers have begun to find ways to improve lipid carriers’ sustainability within a given environment while also increasing target-directed specificity. To achieve better selective targeting by PEG-coated liposomes or other particulates, targeting ligands were attached to nanocarriers via the PEG spacer arm, so that the ligand is extended outside of the dense PEG brush, excluding steric hindrances for its binding to the target receptors [23]. With the increasing momentum behind lipid carrier applications for drug and gene therapy, in addition to limited toxicity and ease of synthesis, contemporary gene therapy may be based in a lipid vector delivery system very soon.
Viral Vectors
With regard to any gene therapy delivery vector, in order to achieve therapeutic success, the transfer vehicles must first be capable of transducing target cells. Second, the administration, transduction, integration, and replication processes should ideally have no impact on nontarget cells. Despite improvements over the last three decades and the most effective transduction methods within gene therapy delivery systems, the viral vectors continue to suffer from a tropism to therapeutic need mismatch [25].
The promise of viral vector-based gene therapy has become integrated within many medical and surgical fields. With tremendous focus and significant accomplishments in both oncology and medical genetics (particularly among those patients suffering from a specific enzyme-deficient pathway), one may ask what is the role in viral vector-based gene therapy in the management of solid organ transplantation or VCA [26]. Heart transplantation and liver transplantation have provided some advances in vector development and target improvement. This has allowed clinical progression within the field of transplantation as realistic and applicable form of therapy for transplant patients . Furthermore, review of the current literature will show how viral vector technology has proved to be better than nonviral vectors at delivering therapeutic genes to cells. With growing interest and ability within the field of viral gene delivery, transplant researchers are beginning to come to a realization of both the benefits and potential risks in viral delivery systems for gene-based therapy in transplantation [26] (Table 18.2).
Plasmid | Oncoretrovirus | Lentivirus | Foamy | Herpes | Adenovirus | AAV | |
---|---|---|---|---|---|---|---|
Genetic material | DNA | RNA | RNA | RNA | DNA | DNA | DNA |
Genetic material packaging capacity | No limitation | 9 kb | 10 kb | 12 kb | > 30 kb | 30 kb | 4.7 kb |
Duration of expression | Transient | Long | Long | Long | Transient | Transient | Long in postmitotic tissues |
Genome integration | Yes | Yes | Yes | No | No | Rarely | Rarely |
Transduction of postmitotic cells | No | Low | Low | High | High | Moderate | Moderate |
Adenovirus and Adeno-Associated Virus
Adenovirus is a member of the Adenoviridae family and defined as a nonenveloped 100-nm-diameter virus with a double-stranded DNA (dsDNA) genome (insertional genetic capacity of approximately 7–8 kb) which in its natural state typically targets membranes within the gastrointestinal, hepatobiliary, and respiratory systems [27]. Of those 51 serotypes endemic throughout the world, typically serotype 2 and serotype 5 are classified as potential vectors for gene transfer . The virus typically utilizes the endocytic pathway and, once within the cell, does not integrate into the host genome but instead remains an episome within the nuclear envelope. Because of this, there is a reduced risk of insertion-associated mutagenesis, which is unfortunately seen with other genome-integrating viral vectors.
There has continued to be significant improvement in the design of the adenoviral vector which has subsequently led to momentum in technologies that increase the utilization and broaden the application of the adenoviruses as a clinically tangible gene therapy vector construct. There are a multitude of features that adenoviral vectors possess which make them forefront candidates for modification strategies in transplant-related gene therapeutics. Among the promising transplant-oriented elements, most adenovirus subtypes (specifically serotypes 2 and 5) are not found to be associated with severe human illnesses. Because of this, serotypes 2 and 5 retain only limited risk for replication-competent viruses, causing acute infectious pathology in patients receiving adenoviral vector-based therapy [28, 29].
Since adenoviral vectors can effectively transduce nonreplicating and replicating cells, unlike many retroviruses which require active replication of cells in order to propagate their delivered transgene and viral life cycle, they offer a variety of options for genetic engineering in the incongruent proliferative phases seen in cells from donor, recipient, and chimeric tissues, seen in allotransplantation. Beyond an ability to transduce cells undergoing transient proliferative variability, the adenoviral vectors can easily provide systems with high-titer production along with an ability to concentrate based on certain tropism characteristics within each serotype. It remains the combination of all of these characteristics which suggests clinical feasibility within VCA and solid organ transplant. A tangible example of adenoviral application within liver transplant is demonstrated with the capability of adenoviral vector serotype 5 to localize to liver parenchyma and concentrate within hepatocytes following intravenous administration. The absolute value of these findings beyond the setting of conventional orthotopic liver transplantation cannot begin to be quantified at this time since the liver, no matter what solid organ or composite allograft, plays vital roles in acute inflammatory processes and disposal of toxic metabolites, which if genetically altered could promote the survival of any transplant element. The insertional genetic capacity of adenoviral vectors is approximately 7–8 kb, and they have high-level transgene expression. The ability of adenoviral vector serotype 5 to localize and concentrate in the liver after intravenous administration in mice allows for the development of in vivo strategies that can have profound systemic effects.
A drawback of the adenoviral vector associated with nongenomic integration is that the transgene expression within a host cell is relatively short lived, despite efficient transduction and high titer levels that can be achieved with the virus making gene transfer more likely. Additionally, direct intravenous delivery of the virus results in nearly 90 % of the vector being degraded, while the immune system, namely T cells, destroy those cells infected with adenoviral particles. Furthermore, the adenoviral serotype used to infect the cells will develop an antibody-derived immune response to protein expressed on the capsid as such antibodies will be directed to the transgene expressed. These antibodies will remain present and prevent further therapy by that specific adenoviral vector.
Concern over the death of a young man in 1999 at the University of Pennsylvania while being a research participant in an ornithine transcarbamylase (OTC) deficiency transgene research study initiated an investigation and report by the National Institutes of Health (NIH) Recombinant DNA Advisory Committee on the assessment of toxicity and safety when using the adenovirus vector for gene therapy [30]. At the conclusion of the report, the NIH committee made recommendations on general safety aspects such as consent, monitoring, and postmortem examination.
Within the field of solid organ transplantation, free tissue transfer, and VCA, the adenovirus has remained a staple in viral vector delivery of genetic material. Researchers have not only shown that interleukin (IL)-10 transgene delivery by a adenoviral to donors can reduce ischemia–reperfusion injury [31], but that adenoviral vectors can also be used to pre-sensitize a patient by targeting host cells (such as the liver) with allo-major histocompatibility complex (MHC) in order to induce pre-allotransplant tolerance [32].
Adeno-Associated Virus (AAV)
The single-stranded DNA AAV was defined over 20 years ago and was administered to the first human subject in 1995 [33]. A member of the Parvoviridae family (genus Dependoparvovirus), these viral subtypes have been found to not produce human infectious disease pathology within clinical applications of gene transfer [34–36]. While similar to the adenovirus and lentivirus vectors in their spectrum of cellular targets, secondary to advantageous tropisms, the AAV harbors a smaller genome and genomic insertion capability (4–5 kb) as well as possesses a requirement for AAV helper viruses for replication and transgene expression within host cells [37].
Members of the AAV group have been shown to be a useful viral vector in clinical trials, with now 11 in the process for approval in humans. Of those, AAV for the cystic fibrosis gene therapy is perhaps the most well-known application using this specific viral vector. Small, approximately 20 nm in diameter, the virus is currently thought to be nonpathogenic. However, one of the most attractive features of this virus is the specificity of genome integration. Although random insertions can occur at low levels, the AAV virus has the consistent ability to integrate at a region on chromosome 19 termed the AAVS1 site [38]. Mediated by the Rep78 and Rep68 proteins (though involve endonuclease, helicase, and transcriptase functions), the AAV virus is able to replicate, integrate, and rescue the provirus from the genome to undergo both latent and lytic infection. Furthermore, with the assistance of a helper virus such as an adenovirus or herpesvirus, the AAV can initiate productive viral infections with long transgene expression. Other than the rep genes, the AAV 4.7 kb genome contains inverted terminal repeat (ITR) regions, open reading frames (ORFs), and a sequence region containing “cap” genes, which code for capsid and structural proteins. Between these two coding regions, the plasmid or gene of interest can be inserted and the virus can be used to deliver a sequence of interest [38].
Among the 11 AAV serotypes defined, the AAV2 or (serotype 2) has developed a natural viral tropism directed toward smooth muscle, neurons, and skeletal muscle, which remain targets of interest in VCA modeling [39]. Furthermore, one of the most difficult challenges in optimizing viral vectors for gene therapy relates to the immune response of the host toward the viral vector. Interestingly, AAV vectors are associated with low immunogenicity and toxicity, resulting in vector persistence and long-term transgene expression, again suggesting a role in VCA [40]. Additionally, although patients can potentially mount an immune response against the input virions, transient immunosuppression during the vector uncoating phase could be sufficient in blocking this response [41, 42].
Retroviruses and Lentivirus
The Retroviridae family or retroviruses has classically been defined as an enveloped virion particle approximately 100 nm in diameter which possess a single-stranded messenger RNA (mRNA) genome. This obligate cellular parasite was identified by its unique ability to transcribe its two copies of identical single-stranded RNA viral genome into dsDNA following delivery of the pre-integration complex after fusion of the virion to the host cell through binding and fusion proteins. The 10-kb genome of a retrovirus commonly contains four coding regions which are seen as ORFs: gag (viral capsid core proteins), pr (proteases for cleavage), pol (polymerase, synthase, and integration), and env (proteins for viral entry into a cell). Integration of the retro-transcribed dsDNA into the host genome defines the provirus form, where it can replicate and be incorporated into the genome of cellular progeny through somatic mitosis or germ line meiosis [4, 6, 43, 44].
Sequence variations of the “env” gene, known as viral tropism, is what determines the ability of envelope proteins of a given virus to bind and fuse with a defined host or target cell [43, 44]. From a research perspective, manipulation and replacement of the env gene allows one to dictate potential targets of an engineered viral vector and is known as pseudotyping. The practice of viral envelope engineering through pseudotyping, or altering the sequence of the viral “env” gene, not only alters the target cell but also can expand the potential target range to multiple cell types.
With a basic understanding of the unique retrovirus life cycle, one can begin to see the underlying set of weakness in this specific vector gene therapy delivery system. First, cells must be actively replicating and/or undergoing transcription for the virus genome (including the therapeutic gene) to propagate. Second, there is no site-specific region within the genome for viral integration of the provirus. This lack of definitive integration is why provirus insertion within an oncogene can lead to neoplasm. Finally, in addition to building an acquired immune response against virions released from host cells, retroviruses are also uniquely sensitive to the c1 protein of the complement cascade, leading to an inability to maintain prolonged transgene expression.
A genus within the Retroviridae family known as Lentivirus is a subclass that contains five documented serogroups ranging from 80 to 100 nm in diameter. Perhaps known best for its primate infectious lentivirus group, which contains HIV1, HIV2, and simian immunodeficiency virus (SIV), the others are bovine, equine, feline, and ovine. Containing an additional six protein coding regions within its genome, the lentivirus not only has the capacity to deliver more viral RNA into a host cell, but the genus also has an advantage over the standard retrovirus in that it can effectively infect nondividing cells and remain functional during long inactive incubation periods. Consequently, these features make them effective at delivering larger sequence gene therapy options in addition to a broader spectrum of cells based on their relative proliferation kinetics.
As early as the 1990s, there were several clinical trials which utilized retroviral vectors as a means of gene transfer. These studies were frequently related to the treatment of inherited monogenetic disorders and AIDS [4, 45, 46]. However, since the optimal retroviral vector targets are those cells, which have higher tendency to undergo division, which permits viral gene incorporation into the host genome, their application to allotransplant gene therapeutics is limited at this time [4, 6]. Consequent to the lower transduction efficiency of the retroviral vectors, less cells will be able to express the delivered gene. Limited relative expression of the transgene makes the pathways involving anti-apoptotic or ischemia/reperfusion less effective targets for retroviral-directed genetic modification, since both pathways require sufficient molecular opposition to prevent their initiating elements from causing irreversible damage to the cell [4–6].
Despite concerns over gene propagation within hypo-proliferative cell and tissue systems, retroviral-directed gene therapy remains a viable option for genetic transfer. Research into the ability of retroviral delivery of immunosuppressive cytokines, where focal accumulation of such cytokines could reduce immune response elements within the graft without altering the systemic profile is particularly appealing. The stable gene expression of retroviral vectors can be viewed as either advantageous or detrimental, depending on the therapeutic goals one is trying to accomplish. In allotransplantation, stable gene expression is likely required for the prevention of acute cellular rejection. On the other hand, stable linear expression of the transgene may not be necessary to prevent ischemia/reperfusion injury seen at the onset following engraftment [4–6]. Because of these fluid and sometimes transient requirements for gene expression, the lentivirus subtype of retroviruses would be required which could propagate transgene expression in “injured” grafts that may not be orienting their metabolic pathways toward cellular proliferation (nondividing cells) but rather toward cell and tissue repair and disposal of toxic products of ischemia such as free radicals, lactic acid, and inflammatory lipid components [47–49].
SI RNAs
Descriptions of relatively short noncoding RNA elements (~25 nucleotides) within cells became relevant in the 1990s, when researchers found them to function as a type of nucleotide sequence-specific defense system which could target both cellular and viral mRNAs for destruction [50]. Small interfering RNA or (siRNA) is a form of dsRNA with the capacity to induce posttranscriptional gene silencing within cells through antisense binding to active form of mRNA and subsequently inducing endonuclease activity against the mRNA transcript/siRNA complex. Since the discovery of this mechanism of RNA interference using siRNA, researchers have begun to develop a spectrum of powerful tools to downregulate mRNA levels and even silence genes involved in the pathogenesis of various diseases associated with a known genetic background [51].
Although there are relatively limited studies on VCA and siRNA applications, the field of solid organ transplantation has developed basic therapeutic constructs and models. Researchers in renal transplantation have sought siRNA technologies therapy directed toward not only rejection but also ischemic–reperfusion injury. In order to prevent ischemia–reperfusion injury and damage to allograft tissues, siRNA technology is being actively studied [52]. However at the current time, most of these attempts at either naked or vector-based siRNA therapy within the arena of solid organ transplantation have been performed in vitro, with very limited studies progressing toward more advanced in vivo settings or animal models. Of the delivery mechanisms employed for siRNA therapeutic application, hydrodynamic intravenous injection of naked or carrier-bound forms of siRNA are most commonly the route for delivery of these RNA interference constructs [4–6, 52]. Of the gene targets thought to be prime targets for siRNA interference therapy, roughly 50 have been tested in transplantation-related models. Most of these mRNA transcripts belong to genes that are either related to apoptosis or involved in immunomodulatory networks. Curiously, secondary to the small size of the interfering RNA, researchers have seen opportunity to combine multiple forms of siRNA in order to downregulate multiple targets. These separate siRNA elements can be transported within the same delivery vector or injected at the same time and by targeting more than one pathway, or by hitting the same pathways within two different key points, will augment the effects of each other [52].
Cells as Gene Therapy Vectors
DST, although discovered over 30 years ago as a potential tool to induce donor-specific immunological tolerance in renal transplant, the protocol has widely been abandoned in contemporary clinical solid organ transplantation. Despite researchers having shown some encouraging results with regard to immunosuppressive drug minimization in human patient subsets and potential induction of immunological tolerance in some animal model systems, the mechanism underlying tolerance induction to recipient tissue remained vague and associative at best [53]. Not until recently did transplant immunologist begin to understand the immunomodulatory mechanisms of DST or donor bone marrow infusion for inducing tolerance, particularly within skin containing VCA. Building on previously published concepts, which indicated that increased levels of regulatory T cells (Tregs) in recipients of an allograft prevented both acute and chronic rejection , investigators then identified the subset of cells dictating the immunomodulatory processes involved. Results of these studies show tolerance is mediated by an interaction among CD11b+expressing cells (DCs, dendritic cells, and macrophages) augmented indoleamine 2,3-dioxygenase (IDO) and IL-10 expression and subsequent Foxp3+CD4+CD25+expressing Treg induction [53, 54].
Transdermal Gene Delivery (Gene Gun)
Described in a letter to Nature in 1987, researchers presented a novel phenomenon, where nucleic acids could be delivered into plant cells using high-velocity microprojectiles through what was described as a gene gun. This and prior research were conducted in the hope of circumventing some of the inherent limitations of existing methods for delivering DNA into plant cells, namely Agrobacterium tumefaciens, which was limited to a specific target host set of plants [55].
The mechanism of delivery utilized small tungsten particles, termed microprojectiles, which where accelerated through a pressurized air gun and used to pierce cell walls and membranes. The microparticles, although penetrating the exterior of the cell, did not kill the organism. Following this success, the microprojectiles were used to carry RNA or DNA into epidermal tissue of an onion for subsequent gene expression analysis.
Since the inception of the gene gun delivery of genes complex with microparticles, the method of delivery has been an option within the search for a more efficient pDNA gene transfer system. Realizing that gene therapy offers a novel approach for the prevention and treatment of a variety of diseases and perhaps a mechanism to promote tolerance in VCA and solid organ transplantation, there remains a paucity of accepted methods because of reoccurring issues concerning either efficacy of delivery or toxicity of vector constructs. Viral vectors, although shown to have higher efficiency of gene transfer, are limited by certain elements of toxicity, host-developed immunity, and length of time related to incubation and transgene expression. While other options of nonviral vector-based delivery systems of pDNA are relatively safe, the efficiency of delivery is significantly lower when compared to viral vector gene delivery [56].
Today, the gene gun, and other similar intradermal delivery devices, takes advantage of the knowledge that skin is known to be a highly immunogenic site for vaccination and other intradermal gene delivery application devices have been developed. Many of these technologies have been shown to administer materials within the skin by noninvasive or minimally invasive techniques. Those platforms utilizing noninvasive methods include high-velocity powder and liquid jet injection, as well as diffusion-based patches in combination with skin abrasion, thermal ablation, ultrasound, electroporation, and chemical enhancers. Additional “minimally invasive” approaches are largely based on microneedle injections [57].
In summary, the choice of a vector to deliver the genetic material of interest is almost as important as what is being delivered. Critical factors include timing, target tissue type, expression level, and safety. The VCA scenario is more challenging due to the variety of tissue types that must be successfully modified, but offers unique opportunities such as the ability to monitor and deliver genes directly through the skin. In general, most groups studying gene therapy in VCA have utilized vectors successfully used for solid organ and systemic gene therapy approaches as we will see below (Table 18.3).
Vectors | Advantages | Disadvantages |
---|---|---|
Retroviruses | Broad cell tropism | Infects only dividing cells |
– | Stable gene expressiona | Risk of insertional mutagenesis |
– | High-titer production (107 cfu/ml) | Susceptible to complement degradation |
– | Large insertion capacity 10 kb | Risk of recombination with human endogenous retroviruses |
– | Low levels of gene expressiona | Risk of competent virus formation |
– | – | Low levels of gene expressiona |
– | – | Stable gene expressiona |
Lentivirus | Broad cell tropism with retroviral and VSV G pseudotyping | Risk of insertional mutagenesis |
– | Can infect nondividing cells | Pseudotyped vectors are susceptible to complement degradation |
– | Stable gene expressiona | Risk of recombination with human endogenous retroviruses |
– | High-titer production (107 cfu/ml) | Risk of competent virus formation |
– | Large insertion capacity 10 kb | Serum conversion with HIV-based vectors |
– | – | Stable gene expressiona |
Adenovirus | High levels of gene expressiona | High levels of gene expressiona |
– | Infect nondividing cells | Transient gene expressiona |
– | Very high titers (1012 pfu/ml) | Immunogenic |
– | Large insertion capacity 8 kb | In vivo delivery minimized by host immune response |
– | Transient gene expressiona | – |
AAV | Infects nondividing cells | Stable gene expressiona |
– | Stable gene expressiona | Requires a helper virus for replication |
– | High titers (1010 cfu/ml) | Small insertional capacity (5 kb) |
– | Nonpathogenic | Risk of insertional mutagenesis |
– | – | Low transduction levels |
Foamy virus | Infects dividing cells | Suboptimally infects nondividing cellsa |
– | Suboptimally infects nondividing cellsa | Stable gene expressiona |
– | Stable gene expressiona | Risk of insertional mutagenesis |
– | Broad cell tropism with retroviral and VSV G pseudotyping | Risk of recombination with human endogenous retroviruses |
– | Large insertion capacity | Risk of competent virus formation |
– | Resistant to complement-mediated lysis | Serum conversion to foamy virus |
Cationic liposomes | Noninfectious | Toxicity dose dependent and most evident in nonreplicating cells |
– | No limit to size of DNA insert | Transient gene expressiona |
– | Transient gene expressiona | Low transduction levels |
– | – | Difficult to implement clinically |
Naked DNA | Noninfectious | Transient gene expressiona |
– | No limit to size of DNA insert | Low transduction levels |
– | Transient gene expressiona | Stimulation of host immune responses due to bacterial CpG |
The Utility of Gene Therapy in VCA and Solid Organ Transplantation
Gene therapy within solid organ transplant or VCA, although not as well understood as the role of gene therapy in cancer, there still remains promise and tangible cell and pathway targets, which within the realm of transplant immunology is well studied. Because of this, many in the field believe that if a proper vector could be developed for delivery, immunosuppression and cellular rejection would be the first targets for genetic alteration.
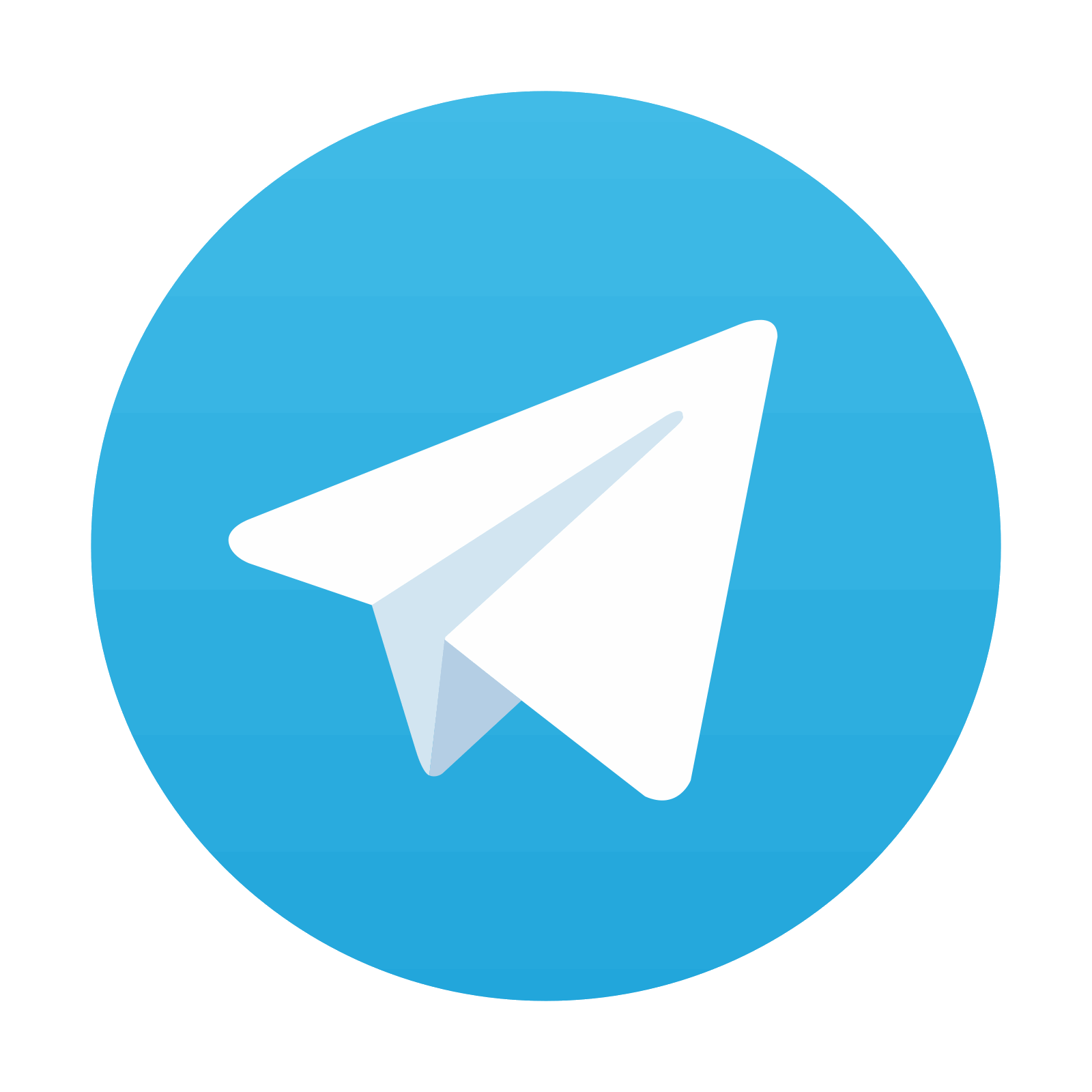
Stay updated, free articles. Join our Telegram channel
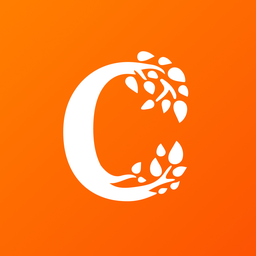
Full access? Get Clinical Tree
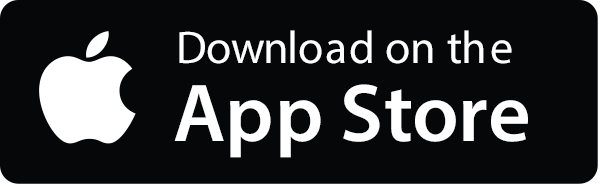
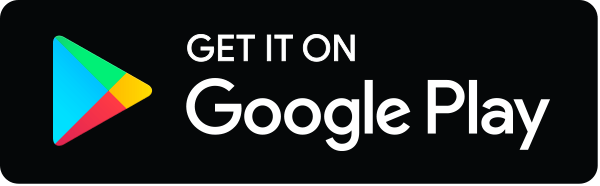