30.2 Intradermal Injection
In present medical practice, most vaccines are administered intramuscularly (IM) or subcutaneously (SC) by hypodermic needle injection. For delivery of vaccines into the dermis region, hypodermic needles are used in a special method called the Mantoux technique. The Mantoux technique is an intradermal (ID) injection method that was developed by Charles Mantoux in the early twentieth century (Mantoux 1909). In this method, a high-gauged hypodermic needle (higher than 25 g needle) is inserted into the skin at a 5–15° angle and 1 mm total depth into the dermis area. When the vaccine is slowly injected into the skin by ID injection, the formation of “blebs” (slight swellings) on the skin can be observed, which can also be significant indicators for successful ID injection. However, this administration method requires highly trained personnel and is considered an inconsistent delivery method due to skin elasticity (Lambert and Laurent 2008). In addition, it is greatly difficult to deliver accurate amounts of vaccines into the dermis region using this technique. Therefore, the development of reliable intradermal vaccine delivery methods has been a compelling issue, regarding which various methods have been investigated globally in recent years (Kim et al. 2012b).
30.3 Ballistic Vaccination: Particle-Mediated Epidermal Delivery (PMED/Gene Gun)
Gene gun is a representative ballistic vaccination method that delivers plasmid deoxyribonucleic acid (DNA) vaccine-coated microbeads, such as gold particles, across the skin and targets skin cells for gene transfection (Fig. 30.2). In this method, DNA-coated particles are accelerated by high-pressured gas to penetrate skin and cell membranes (Yang and Sun 1995). This approach was originally developed as a gene transfer tool for plant cells (Klein et al. 1987) and was also applied for animal DNA vaccination (Fynan et al. 1993).


Fig. 30.2
Gene gun device (This figure was modified and redrawn from following webpages (http://www.bio-rad.com/prd/en/US/LSR/PDP/42e9d6be-369a-49f8-8fbb-281a0fea6df8/Helios-Gene-Gun-System, http://www.1cro.com/campbell/hottopics/dnavaccines/dnavaccines.html))
Subsequently, ballistic vaccination was applied in numerous studies of various disease models. When a DNA vaccine for protecting against Helicobacter pylori was delivered by gene gun in a mouse model, with interleukin-2 (IL-2) and B subunit heat-labile toxin gene as adjuvants, strong immune response and great reduction in bacterial load were observed (Chen et al. 2012). Similarly, strong antibody responses and survival rates were reported when a DNA vaccine against Japanese encephalitis virus infection formulated on chitosan nanoparticles was administered by this method (Huang et al. 2009a). In addition, human papillomavirus (HPV) was targeted by DNA vaccination using gene gun, which resulted in strong CD8+ T-cell immunity, albeit inferior to that elicited by HPV pseudovirions, which are composed of papillomavirus capsid proteins (Peng et al. 2010). It was also reported that a DNA vaccine based on single-chain major histocompatibility complex (MHC) I trimer against Listeria monocytogenes infection was delivered by gene gun, which activated CD8+ T-cell immunity (Kim et al. 2012a). Furthermore, cattle were immunized by gene gun with a DNA vaccine against bovine herpesvirus infection. In this study, mucosal immunization delivered intravulvomucosally showed stronger humoral and protective immune response than intradermal delivery (Loehr et al. 2000). Brugia malayi-abundant larval transcript-2 (BmALT-2) DNA vaccine was delivered using gene gun and was compared with conventional intradermal injection. It was asserted that gene gun methods provided significant protection against challenge and significant dose sparing (Joseph et al. 2012). In another study, it was shown that allergy response was prevented by the delivery of DNA vaccines encoding the birch pollen allergen (Bet v 1) and the grass pollen allergen (Phl p 5), but lung eosinophilia was promoted by gene gun immunization (Scheiblhofer et al. 2007).
Influenza virus is the most well-studied disease model in the field of DNA vaccine research. Influenza DNA vaccine delivery by gene gun was compared with intramuscular or intradermal injection (Feltquate et al. 1997) and electroporation (Wang et al. 2008). When compared with IM or ID injection, gene gun showed different T helper (Th) cells and antibody responses. Gene gun delivery induced Th2 response with a predominant production of immunoglobulin G1 (IgG1) antibodies, whereas IM or ID injection raised Th1 response with a higher ratio of immunoglobulin G2a (IgG2a) antibodies. This trend of immunity was confirmed in CpG motif DNA vaccination studies (Weiss et al. 2002). In addition, when electroporation was compared with gene gun, similar immunizing effects, but different Th cell responses, were observed (Wang et al. 2008).
Ballistic vaccination was also extensively applied in Alzheimer’s disease studies. The first immunization study using amyloid beta 42 (Aβ42) peptide DNA vaccine elicited strong antibody response but did not derive cellular immunity (Qu et al. 2004). The following studies presented that prophylactic Aβ42 DNA vaccination reduced the deposition of Aβ42 peptide in the brain and derived Th2-polarized immune response (Qu et al. 2006, 2007). More advanced immunization studies using gene gun with DNA β-amyloid1-42 trimer immunization were compared with conventional intraperitoneal vaccination with Aβ42 peptide and Quil-A adjuvant. Gene gun immunization with DNA β-amyloid1-42 trimer correlated with IgG1-biased immunity, indicating lower inflammation (Lambracht-Washington et al. 2009). In recent studies, more comparative analyses between various plasmid systems were carried out (Qu et al. 2010). Safety tests for gene gun immunization with DNA β-amyloid1-42 trimer confirmed that this immunization method caused less inflammatory responses due to the lack of T-cell proliferative response and interferon-gamma (IFN-γ) or interleukin 17 (IL-17) cytokine secretion (Lambracht-Washington et al. 2011).
In addition to antigen delivery by gene gun, several vaccine adjuvants were also administered by the ballistic vaccination method. Using gene gun delivery, complement fragment C3d was tested as an adjuvant against malaria and derived controversial effects with immune-stimulating as well as immune-suppressing effects (Weiss et al. 2010). And avian interleukin 1β (IL-1β) and 6 (IL-6) were tested as potential genetic adjuvants to produce immunoglobulin Y (IgY) antibody response for immunization of laying hens (Niederstadt et al. 2012).
The gene gun method was also applied in DNA vaccination against tumors. In one study, gene gun delivery was compared with biojector and IM injection (Trimble et al. 2003). DNA vaccination with gene gun displayed the most potent regimen for DNA administration, such as the highest CD8+ T-cell concentration and antitumor response. In a different study, gene gun was compared with ID, IM injection, and IM injection combined with electroporation. Gene gun delivery produced less effective results than IM with electroporation, although it must be taken into account that the delivered dose by gene gun was 25 times less than that for IM with electroporation (Smorlesi et al. 2006).
Ballistic vaccination has been tested in numerous clinical studies in various disease models. Phase I human clinical trials were conducted to evaluate the safety and immunity of a DNA vaccine encoding hepatitis B virus antigen in several medical centers organized by PowderJect Vaccine Inc. When a low dose of DNA vaccine (0.25 μg) was administered by gene gun, two immunization regimens could derive a high immune response, but a single immunization could not induce any immunity (Tacket et al. 1999). In a second trial, a higher dose (>1 μg) was used, and three immunizations were applied. From this trial, it was found that gene gun DNA vaccination derived not only humoral immunity, such as antibody secretion, but also cellular response, such as secretion of IFN-γ and cytotoxic T lymphocytes (CTL) response (Roy et al. 2001). In a third study, DNA vaccine by gene gun derived strong seroprotective antibody response, even from human subjects who displayed poor immune response to conventional subunit vaccines (Rottinghaus et al. 2003). In addition to hepatitis B, DNA vaccine against Hantaan virus and Puumala virus was clinically tested in phase I clinical trial and derived safe and strong immunity, as observed by the presence of neutralizing antibodies, which are the critical correlate of protective immunity (Boudreau et al. 2012).
30.4 Epidermal Powder Immunization (EPI)
Epidermal powder immunization (EPI) uses high helium gas pressure as a motive power to deliver vaccines across the skin like the gene gun method (Fig. 30.3). EPI usually delivers powder-formulated vaccines (Chen et al. 2000), but also delivers particle-attaching gene-based vaccines or protein vaccines (Chen et al. 2001b, 2002a).
EPI has shown efficacy in various immunization studies. When influenza vaccine was delivered by EPI, EPI derived not only higher humoral immune responses such as IgG and hemagglutination inhibition (HAI) titer but also better protective immune responses, such as decreased lung virus titer and increased survival rate than those in comparative subcutaneous injection (Chen et al. 2000). Chen et al. also found that EPI could induce mucosal immunity compared with that in subcutaneous injection (Chen et al. 2001a). A synthetic oligodeoxynucleotide containing immunostimulatory CpG motifs (CpG DNA), non-toxic B subunit of cholera toxin (CTB), E. coli heat-labile toxin mutant protein (LTR), and QS-21 were delivered by EPI and evaluated as adjuvants for influenza vaccine in mouse models (Chen et al. 2001a, 2002b, 2003). EPI was also tested in a monkey model in a study which demonstrated that EPI was an efficient immunization tool for nonhuman primates (Chen et al. 2003). A phase I human clinical trial was carried out to evaluate the efficacy of powdered influenza vaccines delivered by the EPI immunization method (Dean and Chen 2004). When compared with intramuscular injection control, EPI derived equivalent or better immunity in seroconversion and geometric mean titers (Dean and Chen 2004). Regarding safety issues, EPI was found to be a safe immunization method based on both systemic and local reactogenicity (Dean and Chen 2004). Chen et al. conducted a mechanism study to characterize the reason behind the superior immunity that EPI displays over other methods, such as IM or SC. They found that Langerhans cells and cytokines from epidermal cells played critical roles in induction of immunity. When the LTR72 adjuvant was delivered together with influenza vaccine by EPI, the adjuvant bound to the keratinocytes of epidermis and resulted in increasing levels of cytokines such as tumor necrosis factor alpha (TNF- α) and interleukin-12 (IL-12) (Chen et al. 2004).
In addition to influenza vaccines, other types of vaccines were tested by the aid of EPI. In the human immunodeficiency virus (HIV) vaccine study, EPI derived antibody responses that were 2 or 3 orders of magnitudes higher than comparative IM control. It was argued that EPI can facilitate target delivery into Langerhans cells (LC) and stimulate LC migration. In this study, the vaccine was a recombinant protein type attached on sugar or gold particle, instead of the powder form of the vaccine itself (Chen et al. 2002a). The targeting of LC by EPI was described in a review article in detail (Chen and Payne 2002). Equine herpesvirus-1 vaccine was also tested in a mouse model using the EPI delivery tool (Kondo et al. 2004).
Air-dried powder form of hepatitis B surface antigen (HBsAg) attached onto gold nanoparticles was delivered by EPI (Chen et al. 2001b; Osorio et al. 2003). When air-dried powder vaccine was delivered, an immune response similar to that stimulated by alum-adjuvanted IM control occurred, but when powder vaccine was co-delivered with CpG DNA adjuvant, an antibody response with a couple order of magnitudes higher was produced (Osorio et al. 2003).
For effective EPI, optimized vaccine formulation was investigated. In this study, spray coating method was suggested for vaccine formulation because the powder form of vaccine should have the following physical characteristics: (1) small size (less than 70 μm) of powder for avoiding injury, (2) narrow particle-sized distribution for uniform acceleration, (3) high density (higher than 1 g/mL) for efficient acceleration by high pressed gas, and (4) maintenance of physical stability (Maa et al. 2004).
30.5 Jet Injector
A jet injector is a needle-free injecting medical device that uses a high-pressurized narrow stream liquid to penetrate across the skin barrier and has been used to deliver various macromolecules such as vaccines (Fig. 30.4) (Mitragotri 2006). This vaccination tool with reusable nozzles was applied successfully globally in the latter half of the twentieth century, and it had significant side effects, such as the risk of disease transmission due to cross contamination. Therefore, newly designed tools have been developed that incorporates more of the advanced technologies of the recent era (Weniger and Papania 2008).


Fig. 30.4
Jet injector device (This figure was modified and redrawn from following reference (Sadowski et al. 2003; Nelson et al. 2004) and webpage (http://www.ameditech.com/medinfo/jet.html))
Historically, jet injectors were used in the smallpox eradication program worldwide in the late 1960s (Foege et al. 1971). In addition to smallpox, jet injectors were used for preventing rabies (Bernard et al. 1987), influenza (Jackson et al. 2001), malaria (Aguiar et al. 2001), hepatitis A (Williams et al. 2000), hepatitis B (Ren et al. 2002), BCG (Parker 1984), measles (Kok et al. 1983), tick-borne encephalitis virus (TBEV) (Omori-Urabe et al. 2011), and many other diseases. In most of these cases, jet injectors showed superior immunity over IM injection that required the use of syringes and needles.
Recently, jet injectors have been actively investigated for the delivery of various DNA vaccines for several diseases. Influenza DNA vaccines were administered by jet injectors into different animal models including mouse, monkey, and pig (Haensler et al. 1999; Gorres et al. 2011). In these studies, jet injectors consistently showed better humoral and cellular immune responses than IM injection. Interestingly, jet injectors were compared with not only IM injection but also the gene gun delivery method in the hepatitis B disease model (Ren et al. 2002). In this study, gene gun showed better gene expression than jet injectors, whereas the immune response was equivalent. Therefore, jet injectors were suggested as a simpler DNA immunization method over gene gun. In the malaria disease model, jet injectors demonstrated advanced immunity over IM injection for DNA vaccine delivery (Aguiar et al. 2001). While most jet injecting studies targeted the skin as the site of administration, the vaginal mucosa has been targeted for injecting DNA vaccines (Kanazawa et al. 2010). In this study, a marker DNA (luciferase expressing cDNA fragment) and a model DNA vaccine (pDNA-encoding ovalbumin (OVA)) were delivered through vaginal mucosa by jet injector, and improved marker gene expression and better mucosal antibody response were observed compared to conventional needle syringe injection.
As mentioned above, jet injectors have been widely used in the prevention of various diseases. Numerous clinical studies documented the safety, tolerability, and immunogenicity of inactivated virus vaccines against influenza that were delivered using jet injectors (Jackson et al. 2001; Simon et al. 2011). Although there were no serious adverse events or local reactions, it was reported that jet injectors caused higher pain compared with IM injection. In case of immunogenicity, jet injectors induced an equivalent immune response compared to needle syringe administration. Similarly, the malaria disease model was assessed in a clinical study for safety, tolerability, and immunogenicity and showed similar results with those of influenza studies (Epstein et al. 2002).
WHO Global Polio Eradication Initiative has expressed high interest in the jet injector method as an alternative for oral delivery of poliovirus vaccine (Kim et al. 2012b). Even though the effective immune response that could be stimulated by jet injectors is still beyond reach of expectations, many clinical studies are underway to eradicate poliovirus disease from earth (Epstein et al. 2002).
Most of the mechanism studies regarding jet penetration into the skin have been carried out by Mitragotri’s group. They found that the penetration of liquid jet into the skin was strongly dependent on jet diameter and jet velocity. Working with an in vitro porcine skin model, this group suggested that the optimum values of diameter and velocity were 152 μm and 150 m/s, respectively (Schramm and Mitragotri 2002). More studies focused on the delivery profile in different models, including human skin (Schramm-Baxter and Mitragotri 2004; Baxter and Mitragotri 2005; Shergold et al. 2006) and skin-mimicking material such as polyacrylamide gels (Schramm-Baxter et al. 2004; Schramm-Baxter and Mitragotri 2004) or silicone rubber (Shergold et al. 2006). In a human skin model, the distribution of delivered model drug by jet injector was visualized in a cross-sectional view analysis. In this analysis, it was found that the depth of jet stream penetration increased with nozzle diameter, and the shape and depth of jet injection depended on jet exit velocity (Schramm-Baxter and Mitragotri 2004). The penetrating depth into the skin was predicted by theoretical analysis and was verified with skin-mimicking polyacrylamide gels (Baxter and Mitragotri 2005). In advanced studies, in order to achieve shallow penetration of jet stream, a pulsed injector system “microjet” was developed (Arora et al. 2007). Compared to the conventional jet injector, microjet can deliver vaccines into the shallow epidermis region, which is replete with Langerhans’ cells (critical skin antigen-presenting cell). Therefore, microjet can derive effective immune response and can be considered as a promising skin vaccination device. In addition, microjet can deliver extremely small volumes of drugs across the skin, so that pain can be reduced and precise delivery can be achieved.
For improved pulsed injecting module, the dynamic control of jet velocity during injection pulse was investigated (Stachowiak et al. 2009). In this study, high speed of jet stream was applied to define the depth of delivery, and lower speed was followed to define drug dose, preventing splash back effect. Microparticles were also delivered by a jet injecting device, and shallow delivery into skin was also obtained by particle delivery. Particle delivery was controlled by particle diameter, standoff distance of the nozzle from the skin, injection volume, and concentration of particles (Michinaka and Mitragotri 2011).
30.6 Tattoo Vaccination
Tattoo guns use high-frequency oscillating needles to make thousands of punctures in the skin, which is conventionally used to deposit tattoo ink in the dermis, but has been adapted to deliver ID vaccines (Fig. 30.1) (Chiu et al. 2012). One of the major advantages presented by tattoo vaccination is the fact that this method, unlike intradermal injection, divides a particular dose of vaccine into smaller subportions to be evenly injected into a larger area of the skin. The result is that the vaccine administration is likely to stimulate more antigen-presenting cells in the upper layers of the dermis and the epidermis while conferring the benefit of vaccine-specific cellular and humoral immune responses (Potthoff et al. 2009). In one study, hemagglutinin-expressing DNA vaccine was administered to pigs and derived humoral and protective immunity, as shown by methods including hemagglutination inhibition (HAI) titer and improved virus clearance from nasal swabbing (Eriksson et al. 1998). To overcome the slow processing of an immune response induced by DNA vaccination, DNA tattooing was suggested for short-interval DNA vaccination (Bins et al. 2005). Based on the observation that tattoo immunization resulted in only transient antigen expression that disappeared after 4 days, it was postulated that repetitive application of DNA in between short intervals could boost the absolute amount of antigen and induce faster vaccine-specific T-cell responses. Hence, a new protocol of 0-, 3-, and 6-day scheme was proposed, as compared to the former standard of three immunizations at 2-week intervals. In this study, it was shown that short-interval ID DNA tattoo immunization generated fast and stable T-cell responses to human papillomavirus and complete protection from influenza virus challenge. When compared to the IM route, DNA tattoo vaccination elicited much stronger and quicker humoral and cellular immune responses. Moreover, the local trauma that is caused by insertion of numerous tattoo needles at the site of application has been related to an acute inflammatory phase that attracts leukocytes, which are involved in cytokine and growth factor release (Gopee et al. 2005). Thus, it was postulated that such ensuing inrush of pro-inflammatory cytokines in the vicinity of application was responsible for the prominent immunogenicity conferred by tattooing technique. In addition, studies indicated that even IM immunization in combination with the adjuvants cardiotoxin and granulocyte-macrophage colony-stimulating factor (GM-CSF) was inferior to DNA tattoo immunization alone (Pokorna et al. 2009). More importantly, it was demonstrated that while cardiotoxin pretreatment or GM-CSF delivery clearly improved the immunogenicity of IM immunization, the adjuvants had no boosting effect on that of intradermal tattoo vaccination. Hence, it was proposed that the tattooing technique itself partially substitutes for the function of adjuvants. Furthermore, to determine the effect of the tattooing process on DNA vaccine stability, the DNA topology change was evaluated, including critical factors for antigen expression and immune response (Quaak et al. 2009). It is known that gene transfer methods such as jet injection or electroporation cause a greater shear stress to plasmid DNA than classical needle injection. Such processes result in degradation of plasmid DNA and cause topological conversion into open circular or linear forms, which exhibit lower transfection efficiency than their intact supercoiled counterpart. Nevertheless, in this study, it was found that the DNA tattooing tool had negligible effect on DNA structure and activity. Other vaccines including an adenoviral vector vaccine against respiratory syncytial virus (Potthoff et al. 2009) and a peptide vaccine against human papillomavirus (Pokorna et al. 2009) were administrated by ID tattooing. In the case of the adenoviral vector vaccine, tattooing showed similar performance to ID injection, without notably enhanced efficacy of DNA transfection or improved immunogenicity as when tattooing was compared to IM vaccination. On the other hand, tattooing of the peptide vaccine with CpG motif adjuvant showed better response than IM vaccination with adjuvant.
DNA tattooing was evaluated in nonhuman primates, which have previously shown poor DNA vaccine immunization effect on account of the “simian barrier,” which relates to the factors responsible for the observed discrepancy between the high efficacy of DNA vaccines in murine models but not in nonhuman primates and humans. Exploiting DNA tattooing for delivery of HIV vaccines in rhesus macaques showed remarkable enhancement of immune response, as much as 10- to 100-fold increase in vaccine-specific T-cell responses as compared to when immunized by IM method (Verstrepen et al. 2008). In order to advance this technique to human clinical trials, a human ex vivo skin model was tested, which showed that DNA concentration was the most critical factor for effective DNA vaccination by tattooing (van den Berg et al. 2009). A human clinical trial for treating melanoma by DNA tattooing is being planned for future studies (Quaak et al. 2008).
30.7 Vaccination Using Microneedles
Microneedles (MNs) are micron-sized needles which can be classified into mainly four groups depending on their topography as solid MNs (SMNs), hollow MNs (HMNs), coated MNs (CMNs), and dissolving MNs (DMNs), respectively (Kim et al. 2009, 2010a, b, c, d; 2011, 2012c, 2013, 2014; Quan et al. 2010).
SMNs have been pretreated to make pores for the delivery of diphtheria toxoid adjuvanted with cholera toxin which was able to induce immune response with that of a subcutaneous vaccination (Ding et al. 2009a, b). A similar approach for the delivery of the influenza vaccine boosted immune response when combined with cholera toxin as an adjuvant (Ding et al. 2009b). Further efforts have been done to have improved immune response with diphtheria toxoid antigen with cationic liposome and anionic surfactant-based vesicles (Ding et al. 2009a). The chemically modified antigen with particulated materials has been found to be immunogenic with excellent delivery efficiency. Ovalbumin-conjugated solid lipid nanoparticles (SLP-NPs) were also delivered transdermally by pretreatment with a roller-type MN that showed higher delivery efficiency compared to the antigen application alone (Kumar et al. 2011). Similarly blunt-tip MNs were used for the delivery of hepatitis B-DNA vaccine (Mikszta et al. 2002), recombinant anthrax vaccine (Mikszta et al. 2005), attenuated Japanese encephalitis vaccine (Dean et al. 2005), and rabies vaccine in human tissues (Laurent et al. 2010). When the efficiency of each vaccination was compared to subcutaneous injection, their effectiveness was lower than that of the subcutaneously injected groups because of inefficient delivery through topical formulations.
Since most SMNs have some limitation such as vaccine loss and low delivery efficiency, scientists have come up with HMNs. HMNs are specifically used for fluid or liquid formulations. The therapeutic formulation can flow through the HMNs with a pressure-driven external force. Since the flow rate can easily be controlled through the external pressure, slow infusion and time-varying delivery rate are possible (Yuen and Liu 2015). The injectable liquid formulation enables simplified injection procedures. The major disadvantages are low drug stability, shelf life, and less patient convenience unlike SMNs, CMNs, and DMNs. The initial reports on HMNs were for vaccine delivery for influenza vaccination in rat skin, showing 100-fold dose-sparing effect by using inactivated virus vaccine and a fivefold sparing effect via DNA vaccine compared to intramuscular delivery (Alarcon et al. 2007). The anthrax recombinant protective antigen was also delivered by intradermal injection using HMNs which could generate similar immune response in both mice and rabbits (Mikszta et al. 2005). An anthrax vaccination was able to induce full protection in an immunized rabbit group, and a 50-fold dose-sparing effect was observed compared to the intramuscular track (Mikszta et al. 2006). Except for influenza vaccination with a HMN, various types of vaccines have been used in intradermal immunization such as Japanese encephalitis in primates (Dean et al. 2005), plague F1-V vaccine in mice (Huang et al. 2009b), and a mixed type of vaccine, which consists of anthrax, botulism, plague, and staphylococcal toxins in rhesus macaques (Morefield et al. 2008). HMN-based vaccination system for influenza vaccine was used in a human clinical trial showing a similar immune response as a decreased dose (6 μg) compared to 15 μg full dose of a protein-based influenza vaccine in 10–60-year-old groups, but showing low immunogenicity in older patients (Belshe 2004). Proper dose of the HMN intradermal injection was confirmed as 9 μg in non-elderly adults. For healthy adults, when 9 μg of influenza vaccine was delivered by intradermal injection, it showed an inferior result in a phase II clinical trial compared to the intramuscular injection group with a full dosage of 15 μg (Leroux-Roels et al. 2008; Beran et al. 2009). Except for non-elderly immunized adults, an elderly group was vaccinated with 15 μg full dose intradermally showing a higher immunogenicity compared to the same dose group vaccinated intramuscularly in phase II and III clinical trials (Holland et al. 2008; Arnou et al. 2009).
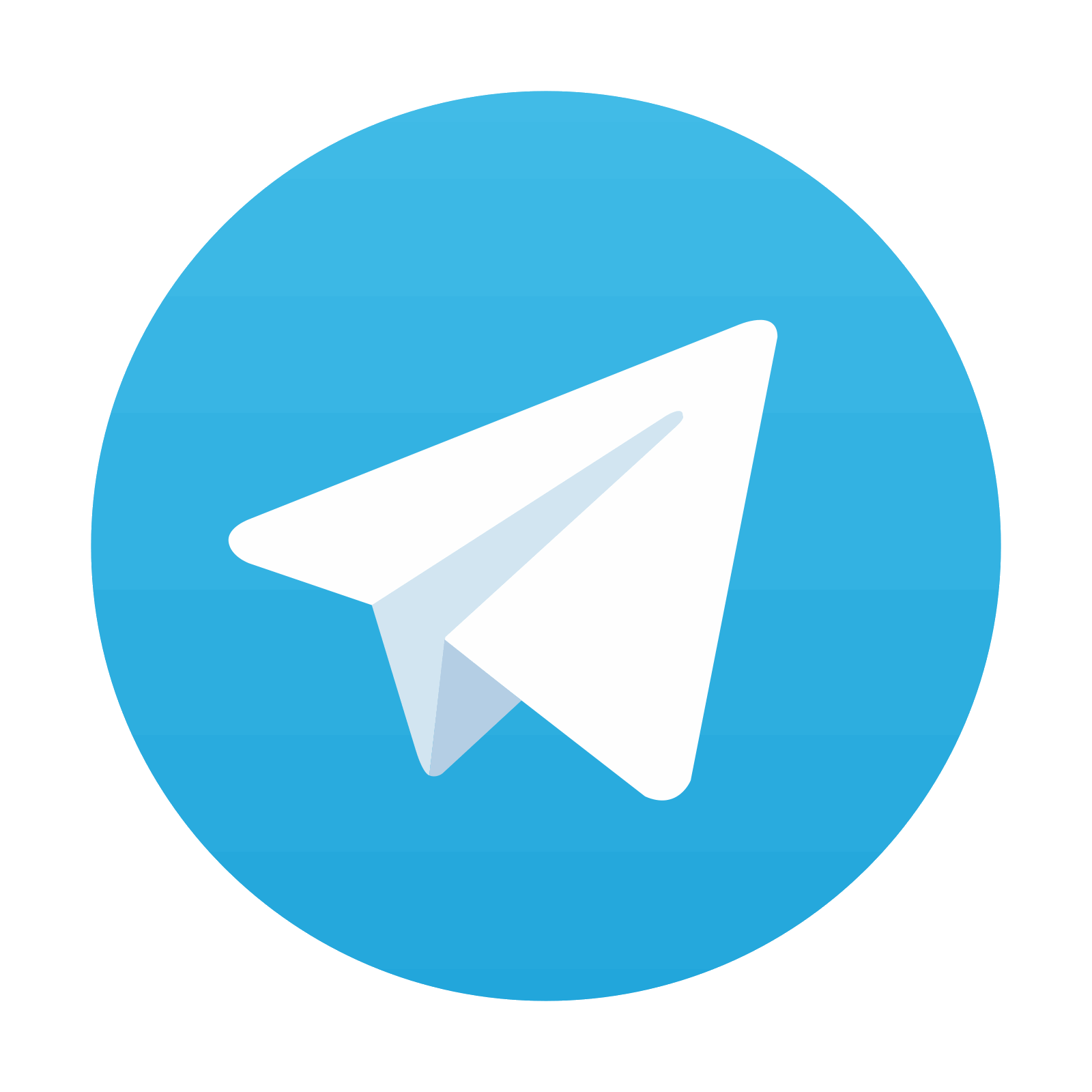
Stay updated, free articles. Join our Telegram channel
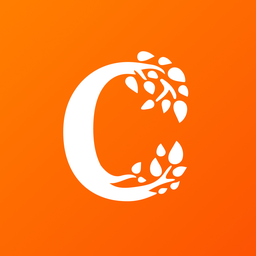
Full access? Get Clinical Tree
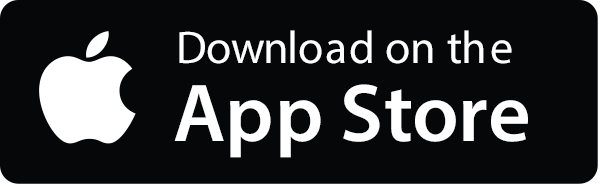
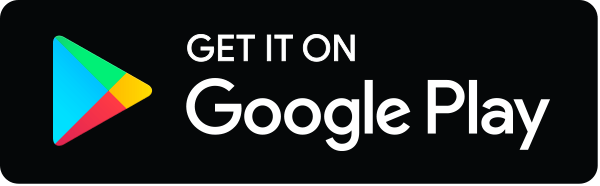