Facial paralysis can have a profound effect on the patient from both an aesthetic and functional point of view. Just as there are numerous etiologies of facial paresis, there are as many therapeutic options and variations of these options. The purpose of this article was to review the most current surgical options for neural reanimation of a damaged facial nerve, including recent advances in nerve repair, conduit technology, and nerve transfers, as well as emerging technology in translational research with biomedical engineering and tissue engineering.
Key points
- •
Review of peripheral nerve anatomy, injury, and repair.
- •
The goal of facial reanimation is to restore facial symmetry and function by primary nerve repair whenever possible, followed by nerve substitution.
- •
There is an explosion of applicable technology related to biomedical and tissue engineering toward facial reanimation.
There is no treatment that can guarantee total recovery and normalization of function following repair of an injured nerve. The poor outcome reflects the complexity of peripheral nerve injuries and the diversity of cellular and biochemical events required to regain function. Facial reanimation has been an area of much research by surgeons, research scientists, biomedical engineers, and tissue engineers due to the devastating impact of facial paralysis aesthetically and functionally. This article focuses on advances in neural reanimation as well as new technologies on the horizon.
This will include discussion of nerve repair, cable grafting, nerve substitution procedures, use of conduits, autografts and allografts, and future directions.
Nerve injury
It is important to review the process of nerve injury and repair when considering therapeutic strategies to simultaneously potentiate axonal regeneration, neuronal survival, modulate central reorganization, and inhibit target organ atrophy. The processes of nerve regeneration and target reinnervation are complex, involving physiologic, biochemical, and cellular changes throughout the whole length of the neuron.
From the moment of injury, the distal nerve stump undergoes Wallerian degeneration, while the proximal stump retracts and the Schwann cells activate nearby macrophages to clear the injured axon.
In addition to debris removal, Schwann cells serve several other functions important for recovery from nerve damage. Whereas these cells normally provide axonal myelin to enhance action potential conduction speed, axon transection causes Schwann cells in the distal end of the transected nerve to switch from a myelination phenotype to a growth-supportive one.
The axon sprouts and a growth cone is formed at the tip of each sprout, interacting with activated and proliferating Schwann cells.
Changes also occur to the proximal neuronal cell bodies in dorsal root ganglia with a shift in protein synthesis from a “signaling mode” to a “growing mode” and protein synthesis switches from neurotransmitter-related substances to those required for axonal reconstruction. The gap at the injury site is aligned by Schwann cells coming from the distal stump to form columns of cells called the bands of Bungner. These columns act as a natural “conduit” to guide and help the regenerating axons reach the end organ. Dedifferentiated Schwann cells upregulate the expression of many regeneration-related elements, such as laminin and collagen, which make up the vital extracellular matrix of the nerve. Regeneration involves contact guidance between the growing axon tip and the Schwann cells lining the tube. The regenerated axon must reinnervate the proper target, and the target must retain the ability to accept reinnervation and recover from denervation-related atrophy. Regeneration rate is approximately 1 mm per day; therefore, more proximal injuries lead to longer denervation periods.
Understanding the complex process of regeneration has spurred research in tissue engineering of natural and artificial conduits and the emerging role of cell-based supportive therapies in nerve repair.
Nerve injury
It is important to review the process of nerve injury and repair when considering therapeutic strategies to simultaneously potentiate axonal regeneration, neuronal survival, modulate central reorganization, and inhibit target organ atrophy. The processes of nerve regeneration and target reinnervation are complex, involving physiologic, biochemical, and cellular changes throughout the whole length of the neuron.
From the moment of injury, the distal nerve stump undergoes Wallerian degeneration, while the proximal stump retracts and the Schwann cells activate nearby macrophages to clear the injured axon.
In addition to debris removal, Schwann cells serve several other functions important for recovery from nerve damage. Whereas these cells normally provide axonal myelin to enhance action potential conduction speed, axon transection causes Schwann cells in the distal end of the transected nerve to switch from a myelination phenotype to a growth-supportive one.
The axon sprouts and a growth cone is formed at the tip of each sprout, interacting with activated and proliferating Schwann cells.
Changes also occur to the proximal neuronal cell bodies in dorsal root ganglia with a shift in protein synthesis from a “signaling mode” to a “growing mode” and protein synthesis switches from neurotransmitter-related substances to those required for axonal reconstruction. The gap at the injury site is aligned by Schwann cells coming from the distal stump to form columns of cells called the bands of Bungner. These columns act as a natural “conduit” to guide and help the regenerating axons reach the end organ. Dedifferentiated Schwann cells upregulate the expression of many regeneration-related elements, such as laminin and collagen, which make up the vital extracellular matrix of the nerve. Regeneration involves contact guidance between the growing axon tip and the Schwann cells lining the tube. The regenerated axon must reinnervate the proper target, and the target must retain the ability to accept reinnervation and recover from denervation-related atrophy. Regeneration rate is approximately 1 mm per day; therefore, more proximal injuries lead to longer denervation periods.
Understanding the complex process of regeneration has spurred research in tissue engineering of natural and artificial conduits and the emerging role of cell-based supportive therapies in nerve repair.
Nerve anatomy
It is also important to understand the structural anatomy of the nerve, which includes mesoneurium, epineurium, perineurium, and endoneurium. The mesoneurium is a connective tissue sheath that suspends the nerve trunk within the soft tissue that contains the segmental blood supply to the nerve. The epineurium is a layer of loose scattered fibroblasts, and adipocytes that defines the nerve trunk and provides mechanical protection. Within the nerve trunk, the perineurium is a multilayered sheath of flattened and densely packed supporting pericytes that surrounds groups of axons, and subdivides the nerve into fascicular bundles. Additionally, the perineurium is the major contributor to nerve tensile strength, serves as a diffusion barrier analogous to the blood-nerve barrier, and contains a latticework for vascular bed. The endoneurium is a loose collagenous matrix within each nerve fascicle that surrounds the individual axons and their Schwann cells ( Fig. 1 ).

The blood supply to the peripheral nerve is a complex vascular plexus fed by radicular vessels in the mesoneurium. Anastomotic connections between epineurial and perineurial plexi occur at various levels in the perineurium and eventually arborize into the network of endoneurial capillaries. This vascular plexus is exquisitely sensitive to tension, as animal studies have demonstrated an 80% decrease in blood flow and irreversible ischemic damage with a 15% increase in nerve tension.
Hence, the development of cable grafts and conduits to provide tension-free coaptation and intact vascularity for successful regeneration. It is important to realize and educate the patient that the preinjury facial form and function is never attainable.
Nerve repair
There are 3 surgical reconstruction strategies: (1) direct repair, in which the proximal and distal nerve ends are sutured back together; (2) nerve grafting, required to bridge a gap between nerve ends; and (3) nerve transfer, when the distal or proximal nerve segment is unusable or missing.
Primary Suture Repair
The basic principles of facial nerve repair have changed little since Bunnell performed the first successful infratemporal coaptation in the late 1920s. Primary tension-free neurorrhaphy of fresh nerve endings remains the gold standard. When a sizable gap exists, stretching nerve endings may impair the vascular supply to the nerve. As the nerve is further stretched, the endoneurial connective tissue may rupture. Tension at the repair site also contributes to increases in fibroblastic activity and scarring.
Epineurial repair is generally favored over fascicular repair. Coaptation of the epineurium is easier, faster, and minimizes internal disruption of the nerve and its blood supply. On the other hand, fascicular repairs would potentially provide more precise anatomic reinnervation. Correct fascicle positioning can be confirmed by the continuity of the nerve’s surface structures, such as blood vessels (vasa nervorum) within the epineurium.
Lubiatowski describes a technique in which epineurium covering the distal stump is rolled back and a 2-mm nerve segment is resected. An epineural “sleeve” is created over the proximal nerve end and is sutured to the epineurium 2 mm proximal to the coaptation site with 2 sutures ( Fig. 2 ). Her study showed faster functional recovery in animals compared with standard epineural end-to-end repair. The epineural sleeve provides a biological chamber for the axoplasmic fluid leakage from transected nerve ends, providing a favorable environment axonal regeneration. Additionally, this technique provides guidance for regenerating nerve fibers, enabling a higher number of axons to reach target organs and prevents neuroma formation.

Tissue adhesive
The minimum number of 10 to 0 nylon sutures to provide a tension-free coaptation is best to minimize scar formation. However, there are still disadvantages with primary suturing related to foreign body reactions to the suture material, excessive handling and manipulation of the nerve ends, trauma from needle penetration through nerve tissue, and difficulty suturing in confined anatomic locations. Subsequently, alternative techniques have been described in published reports for peripheral nerve repair, including gluing, grafting, and laser welding.
Fibrin adhesives (FA) are derived mainly from plasma that facilitates the growth of collagen-producing fibroblasts. Adhesives are easily applied, involve less tissue handling and consequent trauma to the nerve ends, and facilitate the coaptation of the nerve stumps without the demands of microsurgery on both time and surgical expertise.
Knox and colleagues found that the only statistically significant difference between traditional suture neurorrhaphy and FA neural coaptation was the time taken to complete the procedure. Given the reduced operative time required, and ease of application, FA may be an acceptable alternative to suture neurorrhaphy for facial nerve repair.
N-butyl-2-cyanoacrylates used as a tissue adhesive were found to induce fibrosis and foreign body inflammatory reaction and retractile fibrosis, often reducing the nerve diameter up to two-thirds in one study, yet histologic animal studies by another group showed no significant difference in nerve regeneration when comparing suture repair versus cyanoacrylate. Starritt and colleagues compared a sutureless method of facial nerve repair using a biodegradable glass fabric with the standard method of microsurgical suture. Both methods of repair were compared with each other and with a normal control group using electrophysiological and morphometric analysis, with the conclusion that glass-wrap entubulation offers an alternative to standard suture repair. The possibility of sutureless neurorrhaphy with biocompatible substances would be ideal, but due to concerns of tensile strength of repair and inflammatory reaction, suture neurorrhaphy remains the gold standard.
Spector and colleagues addressed the question whether one should mobilize the nerve extensively to achieve end-to-end repair with a single anastomosis, or eliminate tension by bridging with a nerve graft, leaving the regenerating axons to find their way through 2 anastomoses. They reported rerouting of the nerve to achieve primary coaptation yielded better outcomes compared with bridging the gap with a nerve graft. Gardetto and colleagues reported an anatomic study with bridging up to 15-mm gaps in the facial nerve by removal of the superficial lobe of the parotid gland and mobilizing the branches of the parotid plexus.
Nerve grafts
Millesi demonstrated in the 1970s, that grafting of an autogenous nerve segment to bridge a nerve defect leads to better clinical results than suturing the 2 stumps under tension, as is still widely accepted. A nerve graft provides an ideal conduit for regenerating axons because it provides a scaffold that contains Schwann cell basal laminae, and, moreover, these Schwann cells produce growth factors. However, it has several disadvantages, including an extra incision for the removal of a healthy sensory nerve, which will result in a sensory deficit.
The most commonly used donor nerves for facial reanimation include sural nerve, lateral antebrachial cutaneous nerve (LCAN), anterior division of the medial antebrachial cutaneous nerve (MACN), greater auricular nerve, and the motor nerve to the vastus lateralis in cases in which anterolateral thigh free flaps are simultaneously harvested. To choose the best autologous nerve graft, a surgeon has to take into consideration the caliber of the nerve to be repaired, length of the defect, and donor site morbidity.
Does Nerve Graft Polarity Matter?
We are taught that it is important to place a nerve graft so that it is oriented in the same functional direction from which it was harvested. That is, the proximal end of the nerve graft should approximate the proximal end of the host nerve, and the distal end of the graft should anastomose with the distal end of the host nerve, so that axonoplasmic flow should be maintained in the same direction and axons are not lost in branches. However, several animal studies showed that reversing nerve graft polarity of a cable graft did not affect nerve regeneration electrophysiologically or histologically.
Is There a Difference Between Motor Versus Sensory Donor Nerve Graft?
Another interesting question that remains to be answered is whether facial nerve regeneration and synkinesis would differ based on motor versus sensory nerve grafting. In a randomized double-blind placebo-controlled trial using a rat model, motor nerve regeneration increased when nerve gaps were grafted with donor motor nerves in comparison with sensory nerve grafts. MacKinnon postulated that there may be a specificity of motor Schwann cells to produce neurotrophins in response to motor neurites. In another study, MacKinnon found improved regeneration with motor grafting may be a result of the nerve’s Schwann cell basal lamina tube size. Motor nerves have larger Schwann cell basal lamina tubes, which may allow more nerve fibers to cross a nerve graft repair. There are occasions in which the opportunity to use a motor nerve, perhaps even vascularized, is possible when anterolateral thigh flap is planned for reconstruction. Future comparisons of patients grafted with motor nerve grafts for facial nerve defects may be possible.
Allografts are nerve grafts harvested from cadavers, which come with the associated risks of immunosuppression. Recently, AxoGen (Burleson, TX, USA) claimed that their allograft named Avance Nerve Graft has no disadvantages related to immunogenicity due to their decellularized and cleansed extracellular matrix. They have an ongoing study, the Ranger Study, with more than 600 nerve repairs enrolled in January 2015.
Non-nervous biological grafts have included artery and vein segments, as well as skeletal muscle autograft. Autogenous vein is an obvious choice of conduit material, owing to its availability, biocompatibility, and ease of harvest. In 1982, Chiu and colleagues presented histologic and electrophysiologic evidence of nerve regeneration through segments of vein used to bridge sciatic nerve gaps in the rat model. The vein walls are resilient enough to act as a barrier against scar ingrowth and have the permeability to allow diffusion of the proper nutrients. They also can provide a mechanical support for the regenerating axonal cone, offering a protected biochemical milieu, away from surrounding tissue.
Nonbiologic Conduits
Because of the unsatisfactory results achieved by the use of natural conduits, attempts were made to develop a better conduit that can support the adhesion, migration, and function of the local cell and can respect as many properties as possible of an ideal nerve conduit, such as the following:
- •
Biocompatibility
- •
Biodegradability
- •
Permeability and porosity
- •
Protection for axonal growth
- •
Adequate size
- •
Adequate flexibility
Along with the development of tissue bioengineering, the past 30 years saw an impressive increase of experimental studies aimed at testing new biomaterials for nerve regeneration, such as decalcified silicone tube, bone tube, nylon fiber tube, and polyurethanes. Most of the conduits the Food and Drug Administration or Conformit Europe approved for clinical use are made of type I collagen, such as NeuraGen, NeuroFlex, and NeuroWrap, but there are also available conduits synthetized of polyglycolic acid and polylactide-caprolactone (Neurotube, Neurolac). The use of a conduit to connect nerve stumps provides an “open” vehicle for modulation of the cellular and molecular environment for nerve regeneration. The addition of Schwann cells, neurotrophic and neurotropic factors can direct the regenerating axons, while the tube protects the nerve from the surrounding tissue. Placing the lacerated ends in each end of a tube instead of trying to match them at the suture site could also leave the axons to find their way more accurately.
Modifications to the common hollow nerve tube have been investigated and include collagen-containing and laminin-containing gels, internal frameworks, supportive cells, growth factors, and conductive polymers.
Future perspectives aim in a combined approach to the regenerating process, focusing not only on a scaffold that can support growing axons but also improve Schwann cell migration and deliver growth-promoting factors inside the lumen.
Nerve Substitution
Nerve transfers are indicated when the main trunk of the facial nerve is damaged or unavailable for grafting but the distal nerve branches and mimetic muscles remain viable. Patients with acquired paralysis who have undergone serial clinical and/or electromyographic testing that has failed to show any functional recovery by 6 months should be considered for a reinnervation procedure before significant motor endplate atrophy. There is a general consensus that the best and most predictable results are achieved when neurotization surgery is performed within 2 years of the palsy, although Conley reported success within 4 years of palsy. Increased denervation time and advancing patient age both negatively affect nerve regeneration and may lead to inferior outcomes of reinnervation procedures.
The most common nerve substitution procedures that will be discussed include the hypoglossal, masseteric, and cross-facial sural nerve grafts. There are 3 basic ways in which the nerve transfers are being increasingly used: direct motor neurotization, babysitter and double innervation techniques, and the innervation of neuromuscular transplants.
Hypoglossal–Facial Nerve
Since its introduction by Korte in 1901, the hypoglossal–facial nerve substitution was widely popularized by Conley and Baker. The facial and hypoglossal nerves have a cortical topographic proximity in the motor cortex making the hypoglossal a sensible substitution.
Unfortunately, sacrifice of the hemihypoglossal resulted in unacceptable functional deficits impeding speech and mastication, as well as mass movement and synkinesis of the hemiface ( Fig. 3 ).
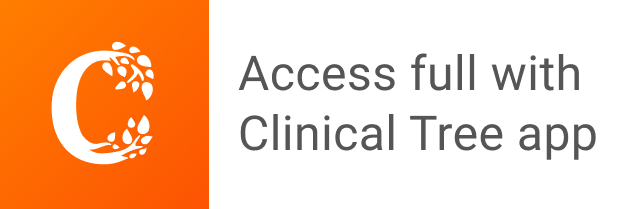