Autologous breast reconstruction is commonly performed after mastectomy and provides a natural replacement that mimics the native breast. Although current free flap success rates exceed 95%, total flap loss can be devastating for patients. As a result, new technologies have emerged to provide an objective means for flap monitoring, with the hope that vaso-occlusive events can be detected before the clinical manifestation of a microvascular complication and the no-reflow phenomenon. This article focuses on the available data for one new technology, near-infrared spectroscopy, and its current use in clinical practice.
Breast cancer is the most common cancer affecting women in North America, and a significant number of these women undergo mastectomy as part of their multidisciplinary treatment. More than 80,000 postmastectomy breast reconstructions are performed in the United States each year, and this rate continues to increase annually. Autologous tissue reconstruction reliably replicates both the appearance and feel of breast tissue while avoiding implantation of a foreign body. However, both free and pedicled flaps are subject to vaso-occlusive events that can lead to flap necrosis. Although the past decade has seen technical improvements in the execution of free tissue transfer, with success rates of 95% or higher, total flap loss from microvascular failure is financially and emotionally stressful for both patient and surgeon. Because salvage rates are inversely proportional to the interval between the onset of vascular compromise and clinical recognition, accurate and timely flap monitoring is critical.
The current benchmark for flap monitoring in autologous breast reconstruction is clinical observation of skin color, turgor, capillary refill, and dermal bleeding. However, more than 90% of surgeons use objective surveillance devices as an adjunct to clinical monitoring. The qualities of an ideal monitoring device, as first described by Creech and Miller in 1975, have remained unchanged. This device should be harmless to the patient and flap, rapidly responsive, accurate, reliable, and applicable to all types of flaps. Despite the rapid progress in technology over the past 3 decades, no instrumentation has been introduced that satisfies all of these criteria. Temperature probes, implantable Dopplers, color duplex sonography, microdialysis, and laser Doppler flowmetry have all been described for monitoring free flaps with varying degrees of accuracy and cost-effectiveness. More recently, a new technology using near-infrared spectroscopy has emerged and shows promise as a clinically useful device for adjunctive monitoring in autologous breast reconstruction. This article focuses on the background, indications, and current clinical data for near-infrared spectroscopy.
Background
Tissue viability depends on adequate oxygen delivery to meet cellular demands. Through evolution, a cardiovascular system developed in multicellular organisms to deliver this essential nutrient beyond the limits of simple diffusion. Because oxygen is poorly soluble in water and plasma, the respiratory pigment hemoglobin evolved to bind oxygen for transport and improve efficiency. Near-infrared spectroscopy uses the selective light absorption characteristics of hemoglobin to provide an assessment of perfusion and tissue oxygen saturation.
Near-infrared spectroscopy measures scattering and absorption of calibrated wavelengths of near-infrared light, which is related to the oxygen content of the hemoglobin within the tissue monitored. Near-infrared light is able to penetrate skin, bone, and muscle to a depth of up to 20 mm with less scatter compared with light of shorter wavelengths. Near-infrared light is emitted from an optode into the tissue and then recovered by a receiving optode. Most frequently, these optodes are placed adjacent to one another with a single probe fixed to the area under investigation. The amount of light recovered from the tissue of interest depends on the intensity of incident light, degree of light scattering, and amount of chromophore absorption, and is subsequently continuously displayed real-time on an external monitor ( Figs. 1 and 2 ).


Chromophores selectively absorb light within tissue variably, depending on changes in oxygenation, resulting in a reduction of light intensity. The principal chromophores in biologic tissues are hemoglobin, myoglobin, and cytochrome c oxidase. In skin, the primary chromophore is hemoglobin, with a small contribution of chromophore by cytochrome c oxidase, and background absorption from other surrounding tissue. Because the degree of scattering can be assumed stable in the same tissue, and the intensity of emitted light is constant, changes in the recovered light can be attributed to the changes in the tissue concentrations of the chromophores. Characteristic wavelengths of optical absorption for both oxygenated and deoxygenated hemoglobin enable both forms to be differentiated and the concentrations quantified.
Near-infrared spectroscopy instruments calculate the ratio of oxyhemoglobin and deoxyhemoglobin to generate a noninvasive real-time measurement of tissue oxygen saturation (StO 2 ). StO 2 is the percentage of hemoglobin bound to oxygen within the volume of tissue underneath the sensor patch. Any hemoglobin in the light path beneath the sensor, whether flowing in vascular space, pooled or extravascular, contributes to the StO 2 . Changes in total tissue blood volume can also be calculated from the summation of concentrations of the oxygenated and deoxygenated hemoglobin, which may be useful in predicting venous insufficiency.
Clinical applications
In 1977, Jobsis was the first to note that biologic materials are relatively transparent in the near-infrared region of the spectrum, permitting photon transmission for monitoring cellular events, changes in tissue blood volume, and the average hemoglobin–oxyhemoglobin equilibrium. Mancini and colleagues validated the functions of near-infrared spectroscopy in humans through measurements of skeletal muscle changes during forearm exercise.
To further determine the accuracy of near-infrared spectroscopy, Myers and colleagues performed a comparison study between near-infrared spectroscopy (InSpectra Model 325, Hutchinson Technology, Hutchinson, MN, USA) and a carbon monoxide–oximeter in a closed circulating blood loop. They found a strongly positive correlation between the two measuring devices at oxygen saturation levels from 5% to 95%, confirming the validity of near-infrared spectroscopy.
Early clinical work with near-infrared spectroscopy was performed in trauma patients. McKinley and colleagues showed the correlation of decreased oxygen saturation measurements with shock and subsequent organ dysfunction. Similarly, decreased near-infrared spectroscopy (InSpectra) measurements of muscle tissue oxygen saturation predicted poor perfusion, multiorgan dysfunction syndrome, and death after severe torso trauma.
With this background of proven efficacy for detecting perfusion and oxygenation, the technology then naturally came under consideration for evaluating thrombo-occlusive events in plastic surgery. Irwin and colleagues used near-infrared spectroscopy (Niro-500, Hamamatsu Photonics, United Kingdom) to measure StO 2 in the hind limb muscles of rabbits with arterial occlusion. They found a rapid decrease in oxygenation with occlusion of the common iliac artery and a rapid increase in oxygenation after restoration of flow.
In humans, Scheufler and colleagues used the device in a pedicled transverse rectus abdominis musculocutaneous (TRAM) flap model. The authors analyzed 11 patients undergoing autologous breast reconstruction with an ipsilateral pedicled TRAM flap. Near-infrared spectroscopy (Multiscan OS10, NIOS-Medical Technologies, Germany) and color-coded duplex sonography measurements were obtained preoperatively, 1 day after surgery, and at late postoperative follow-up after 6 months. Zone IV was discarded in all reconstructions and not included in the measurements. Near-infrared spectroscopy was able to detect preoperative and postoperative differences of tissue hemoglobin content and oxygenation in the TRAM flap, and correlated with clinical examination and color-coded duplex sonography in detecting venous congestion in zone III. Because there was no partial or total flap loss in their series, they were not able to characterize critical thresholds for near-infrared spectroscopy in flaps with impaired perfusion that would result in subsequent necrosis.
The authors initially used near-infrared spectroscopy (ODISsey Tissue Oximeter, ViOptix, Inc, Fremont, CA, USA) to monitor free tissue transfer in an initial study of digit replantation in 48 patients and 64 digits. At the time, serial quantitative fluoroscopy was considered the preferred objective monitor at the Buncke Clinic as an adjunct to clinical examination, but the monitoring was cumbersome and time-consuming, and therefore the authors sought alternative options. No significant differences in StO 2 measurements were seen between the digits that survived (61 digits) and the control digits. Three digit replantations failed. These failed digits had 30% to 70% lower StO 2 values than control digits. This finding correlated with serial quantitative fluoroscopy measurements. The authors concluded that near-infrared spectroscopy was able to accurately detect digit failure and that the technology may be valuable in monitoring digit replantation.
In a second series, the authors obtained intraoperative and postoperative tissue oxygen tension measurements with near-infrared spectroscopy in seven consecutive free flaps performed for breast reconstruction. Measurements were taken at baseline, after flap elevation, with clamping of inflow vessels, after vessel anastomosis, after flap insetting, and continuously during postoperative recovery. Baseline tissue oxygen tissue measurements averaged 83% (range, 70%–99%), which is expected given the mixed oxygen saturation levels of capillary blood. When the vessels were clamped for flap transfer, StO 2 dropped an average of 33% (range, 25%–38%), but rapidly recovered to near preclamping values within 5 minutes after reanastomosis, and measurements remained stable for the next 48 hours ( Fig. 3 ). Although no thromboembolic events occurred in this series, the intraoperative near-infrared spectroscopy measurements correlated with the iatrogenic surgical discontinuation of the blood supply, suggesting that near-infrared spectroscopy may be valuable for monitoring free flaps postoperatively.
Repez and colleagues prospectively evaluated 50 free flaps used for autologous breast reconstruction in 48 patients by using continuous-wave near-infrared spectroscopy (InSpectra, Hutchinson Technology) for 72 hours of postoperative monitoring. In their study, 10 flaps (20%) developed 13 anastomosis thromboses (2 arterial and 11 venous). Near-infrared spectroscopy detected an abrupt decrease in StO 2 for arterial thromboses in all cases of flow failure before clinical evidence of vascular compromise, with no false-positives or false-negatives. Similarly, StO 2 levels dropped with venous occlusion, but the change was more gradual. The flap salvage rate was 70%, with an overall flap viability of 94%. The authors advocate near-infrared spectroscopy for monitoring flaps with a cutaneous component.
Keller reviewed his initial experience with near-infrared spectroscopy (T.Ox Tissue Oximeter, ViOptix) in 30 patients undergoing autologous tissue perforator free flap breast reconstruction. In his series, the device detected two episodes of venous thrombosis before clinically apparent, which allowed for early flap salvage in both cases.
In a second larger series, Keller reported his outcomes in 208 autologous free flaps for breast reconstruction in 145 patients monitored with near-infrared spectroscopy intraoperatively and for 36 hours postoperatively. Five patients had complications that were predicted by near-infrared spectroscopy before any clinical signs, allowing for expeditious flap salvage. The eight reoperations resulted from hematomas, venous congestion, and arterial thromboses. No flap losses occurred in the series. Based on his experience with these thromboembolic events, Keller devised a diagnostic algorithm for early prediction of vascular compromise based on an absolute StO 2 number and the StO 2 drop rate. In this study, a change in StO 2 of 20% or greater per hour predicted a vascular complication. In addition, an absolute drop of StO 2 to less than 30% was also predictive of vascular compromise ( Figs. 4 and 5 ).
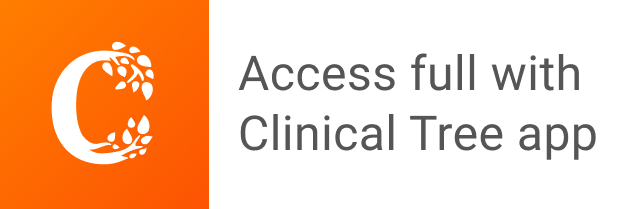