Fig. 18.1
Approaches aiming at facilitation of peptide/protein transport across the skin
This chapter will focus on the use of microneedle (MN) arrays as medical devices to disrupt the barrier properties of the SC to enable enhanced transdermal drug delivery, focusing on the materials used for device manufacture, the forces required for successful MN insertion and the potential safety aspects that may be involved with the use of MN devices.
18.2 Microneedle Arrays
MN technology entails the use of micron-sized needles (microprojections) typically between 100 and 3000 μm in length. They are more commonly between 250 and 1500 μm with a variety of different shapes attached to the base support (Pettis and Harvey 2012). Application of such MN arrays to biological membranes can create transport pathways of micron dimensions (Kaushik et al. 2001). Once created, these micropores, which are predominately aqueous pathways, are orders of magnitude larger than molecular dimensions and, therefore, should readily permit transport of macromolecules as well as possibly supramolecular complexes and microparticles (Prausnitz 2004). In addition, MNs could also be used for the sampling of bodily fluids, such as for measuring the blood glucose levels in diabetic therapy. ALZA Corporation appears to be the first to conceive the potential use of a MN array for transdermal applications (Gerstel and Place 1976). However, it was not until the 1990s, with the advent of high-precision microelectronic industrial tools, that it became possible to successfully produce such microstructure devices. The first report to demonstrate MNs for transdermal delivery was not published until two decades later (Henry et al. 1998).
The major advantage offered by MN technology, in comparison to the aforementioned SC disruption techniques, is its simplicity of use and low cost. MNs allow for easy and patient-friendly administration of therapeutics to and across the skin. Controlled drug deposition within targeted skin layers can be achieved by modulating MN geometry. In addition, simple alteration of drug formulation facilitates optimisation of drug delivery in order to obtain the best therapeutic effect. MNs have been shown to penetrate the skin, across the SC and into the viable epidermis, avoiding contact with nerve fibres and blood vessels that reside primarily in the dermal layer. The basic concept of a polymer MN is imaged below (Fig. 18.2).


Fig. 18.2
Concept of a polymer MN array that can penetrate through the stratum corneum (Reproduced with permission from Elsevier, Ami et al. (2011))
Therefore, the use of MNs provides pain-free delivery for both small and large-molecular-weight APIs and prevents bleeding at the application site (Bal et al. 2008; Pettis and Harvey 2012). Over the last decade, extensive research has been carried out concerning microneedle design with the use of a wide range of techniques and fabrication methods (Pettis and Harvey 2012). Importantly, enhancement in the delivery of drugs and biomolecules of a wide variety of physicochemical properties has been demonstrated in in vitro, ex vivo and in vivo experiments using a broad variety of device designs.
Delivery of therapeutics across the skin with the aid of MNs can be achieved via four main strategies as illustrated in Figs. 18.3 and 18.4. The first approach, termed ‘poke with patch’ involves application of a solid MN array to create micropores. The array is then removed, and the drug formulation is administered in the form of a transdermal patch, gel or solution (Fig. 18.3a) (Martanto et al. 2004; Donnelly et al. 2008). Movement of molecules through microchannels occurs via passive diffusion or if electric field is applied via iontophoresis. Another strategy, ‘coat and poke’, relies on coating a drug formulation onto the microprojections and subsequent insertion of the coated MN array into the skin (Fig. 18.3b). Drug deposition occurs through the dissolution of the coating after being applied to the skin (Gill and Prausnitz 2007a, b). The third mode of drug delivery via MNs utilises incorporation of drug molecules into the structure of polymeric, biodegradable MNs and subsequent insertion into the skin (Fig. 18.3c) (Lee et al. 2008; Park et al. 2006). Drug delivery depends on the rate of polymer dissolution or degradation within the skin. Lastly, drug molecules can be transported across the skin via injection through hollow MNs (Fig. 18.3d) (Davis et al. 2005). Delivery of molecules with the aid of hollow MNs can be pressure or electrically assisted.



Fig. 18.3
Schematic image of drug delivery using different designs of microneedles: (a) Solid microneedles for permeabilising skin via formation of micron-sized holes across the stratum corneum. The needle patch is withdrawn followed by application of a drug-containing patch. (b) Solid microneedles coated with dry drugs or vaccine for rapid dissolution in the skin. (c) Polymeric microneedles with encapsulated drug or vaccine for rapid or controlled release in the skin. (d) Hollow microneedles for injection of drug solution (Reproduced with permission from Elsevier, Shah et al. (2011))

Fig. 18.4
A further schematic representation of the methods of drug delivery into the skin using MNs. MNs are first applied to the skin (a) and then used for drug delivery (b). Solid MNs are used as a pretreatment, after which drug can diffuse through residual holes in the skin from a topical formulation (solid MN). After insertion of drug-coated MNs into the skin, the drug coating dissolves off the MNs and into the aqueous environment of the skin (coated MNs). Drug-loaded MNs are made of water-soluble or biodegradable materials encapsulating drug that is released into the skin upon MN dissolution (dissolving MN). Hollow MNs are used to inject liquid formulations into the skin (hollow MN) (Reproduced with permission from Elsevier, Kim et al. (2012))
18.2.1 Solid MNs
MNs have been fabricated from silicon, metal polymers and less traditional materials such as carbohydrates (Sivamani et al. 2007). The first solid MNs were fabricated from silicon using microfabrication technology (Henry et al. 1998). Other research groups also employed microfabrication methods to produce solid MNs of varying shapes, heights and densities from silicon (McAllister et al. 2003; Wilke et al. 2005; Xie et al. 2005). The fabrication of MNs from silicon via microelectromechanical system (MEMS) technique employs an etching process, either wet (solution) or dry (vapour phase) to selectively remove predefined areas of silicon surface from a flat wafer to create small three-dimensional (3D) structures (Coulman et al. 2006). Although the manufacture of silicon MNs using microfabrication methods offers the potential for mass production of MNs, it is often expensive and highly specialised and includes complex multistep processes (Zafar et al. 2004; Ziaie et al. 2004). Apart from silicon MNs, metal MNs are used. Although they have been found to be equally effective in skin penetration, they are less expensive to fabricate and hence more advantageous. Metals, such as stainless steel and titanium, have been used as structural materials for solid MN fabrication (Cormier et al. 2004; Verbaan et al. 2008). Approaches, such as photochemical etching (titanium) and laser cutting (stainless steel), have been investigated for fabricating solid metal MNs. Metals, such as stainless steel (e.g. hypodermic needles), have been in medical use for decades. Essentially, the use of such materials will effectively reduce the regulatory path of approval, compared with that required for non-approved materials, such as silicon (Mikszta et al. 2006).
A plethora of studies demonstrated the usefulness of solid MNs to increase transport of molecules varying in physicochemical properties into and across the skin. Donnelly et al. (2009a) demonstrated MN-enhanced cutaneous delivery of a model preformed photosensitiser both in vitro and in vivo. The meso-tetra (N-methyl-4-pyridyl) porphine tetra tosylate (TMP, MW = 1363 Da)-loaded patches were applied to MN-treated (6 × 7 silicon MN array, 270 μm in height) full-thickness porcine skin in in vitro experiments and to nude mice in in vivo studies. The results indicated that appreciable amounts of TMP were localised within the skin pretreated with MNs after 6-h patch application time, both in vitro and in vivo, in comparison to the intact skin. The authors concluded that MN technology can find application in topical photodynamic therapy of skin tumours.
Successful intradermal vaccination was also demonstrated after skin pretreatment with solid MNs. Ding et al. (2009) investigated immune responses in mice after MN-mediated transcutaneous immunisation using a model antigen, diphtheria toxoid (DT). Stainless steel MN arrays (4 × 4, 300 μm in height) were used to pierce the mouse skin, and DT formulation with or without cholera toxoid as adjuvant was administered. The application of DT on untreated skin resulted in low serum IgG titres. However, MN pretreatment led to significantly higher serum IgG titres, which were further increased in the presence of cholera toxoid. In a follow-up study, the same research group investigated MN-assisted transport of DT formulations with N-trimethyl chitosan (TMC) as adjuvant into mice skin. The formulations tested were TMC nanoparticles loaded with DT, liquid mixtures of TMC and DT and DT solution alone. It was demonstrated that application of DT solution to MN-pretreated skin elicited stronger immune response in comparison to the administration of DT solution to untreated skin. Administration of DT-loaded TMC nanoparticles did not result in further enhancement of immune response. However, when TMC/DT solutions were applied to MN-treated skin, an eightfold increase in IgG titres was observed in comparison to the application of nanoparticles and DT solution alone (Bal et al. 2010).
The utility of solid MNs to enhance cutaneous gene delivery has also been studied by Pearton et al. (2007), utilising an array of frustum-shaped silicon MNs (with 4 × 4 array, 260 μm in height). It was confirmed that MN application created micropores of approximate depth of 150–200 μm and increased the transepidermal water loss (TEWL). Using this method, the ability of the solid MN arrays to disrupt the skin barrier was assessed in a study in 2002. Two hydrogel formulations loaded with plasmid DNA (pDNA): 1 % w/v Carbopol® 940 and 23 % w/w tri-block copolymer PLGA-PEG-PLGA were applied to the split-thickness human breast skin prior to MN array application. It was demonstrated that functional pDNA was released from both hydrogels in the epidermis. No gene expression was observed after administration of pDNA hydrogels to the intact skin.
Apart from cutaneous delivery, solid MNs have been extensively investigated, as a tool to improve the transdermal delivery of compounds. Super-short silicon MNs (70–80 μm in height) with sharp and flat tips (Fig. 18.5) were fabricated, and their ability to enhance the transport of a model compound, galanthamine (GAL), across full-thickness rat skin was studied (Wei-Ze et al. 2010). The authors evaluated the effect of different parameters, such as MN insertion force (1, 3, 5, 7 and 8 N/array), tip sharpness (sharp and flat tip) and MN density (8 × 8, 10 × 10, 12 × 12) on skin permeation of GAL.


Fig. 18.5
Super-short MNs: (a) a single sharp-tipped MN and (b) a single flat-tipped MN (Reproduced with permission from Elsevier, Wei-Ze et al. (2010))
It was demonstrated that application of forces in the range between 1 and 5 N/array resulted in an increased transdermal GAL transport. However, a further increase in the insertion force beyond this range did not lead to any further permeation enhancement. This indicated that insertion force has a significant effect on the transport of molecules only when complete MN penetration has not been achieved. GAL permeation was increased to a greater extent after skin pretreatment with flat-tipped MNs in comparison to sharp-tipped MNs. In addition, the results revealed that increasing the number of MNs per unit area resulted in increased flux of GAL. However, there was no statistical difference in the cumulative amount of GAL permeated across the skin treated with 10 × 10 and 12 × 12 MN array.
McAllister et al. (2003) provided a comprehensive report demonstrating the efficacy of solid silicon MNs in transdermal delivery of relatively small (calcein) as well as macromolecular (insulin, BSA and nanoparticles) compounds in vitro. Two different scenarios were investigated. Firstly, MN array (400 needles, 150 μm in height) was inserted into human cadaver epidermis and left in place during application of drug solutions. The results showed that skin permeation of calcein, insulin and BSA was enhanced by orders of magnitude. In the second set of experiments, MN array was used to pierce the skin and was removed, after which drug formulations were applied. It was found that skin permeability to model compounds was increased by an additional order of magnitude in comparison to the first scenario. Furthermore, pretreatment of the skin with MNs resulted in the transport of detectable amounts of nanoparticles across the skin. Martanto et al. (2004), from the same research group, investigated the delivery of insulin to diabetic rats using solid stainless steel MNs (105 needles, 1000 μm in height). The effect of insulin concentration (100 and 500 IU/ml), MN array insertion time (10 s, 10 min and 4 h) as well as number of MN array insertions (one insertion and five insertions) on insulin delivery was studied. It was found that higher insulin concentration resulted in greater hypoglycaemic effect. Furthermore, increased MN insertion time led to the smaller reduction in blood glucose levels, which was attributed to the blockage of microchannels by MN array left in situ. Moreover, single insertion of MN array was found to be more effective in reducing blood glucose level than multiple insertions of MNs. The authors hypothesised that this phenomenon was due to local damage of the skin which changed insulin clearance and its absorption by capillary bed. In addition to in vitro and in vivo studies conducted in animal models, Prausnitz and collaborators carried out the first clinical study in human subjects to demonstrate the MN-based transdermal delivery of an opioid blocker, naltrexone (NTX). The choice of this compound was due to its properties – passive transdermal delivery of NTX is limited by its hydrophilic nature, making it a candidate for MN administration (Pettis and Harvey 2012). The skin on the upper arm of healthy volunteers was pretreated with two solid stainless steel MN arrays (5 × 10 MNs, 620 μm in height) which was followed by NTX patch application and collection of blood samples over 72 h. Results showed variable absorption of NTX (1.6–8.1 ng/ml) achieved within wide timeframe (1.5–18 h) of patch application, followed by steady-state plasma concentrations of ~2.5 ng/ml maintained for at least 48 h. Application of NTX patches to untreated skin did not result in detectable drug plasma concentrations (Wermeling et al. 2008). Therefore, this study demonstrates the feasibility of using MNs to enhance transdermal delivery of hydrophilic medication (Pettis and Harvey 2012).
18.2.2 Coated MNs
Coated MNs constitute another mode of MN application, especially attractive for rapid delivery of active substances into the skin. From this, the use of a MN patch, either coated with or encapsulated drugs, is arguably the simplest method of MN-based drug delivery for patients (Kim et al. 2012). Drug formulation is directly coated onto microprojections, and following their insertion into the skin, the drug is released by subsequent dissolution of the coating (Gill and Prausnitz 2007a, b). Coating MN arrays with the drug eliminates the need for two-step application process, which is advantageous over ‘poke and patch’ approach. Also, the desired dose of the drug is delivered into the tissue quickly upon insertion of the MNs. However, a disadvantage of this approach is that the amount of drug that can be coated onto MNs is limited by the small surface area of the array, which in turn, limits drug delivery. For the coated MNs to be a viable option of drug administration in clinical setting, uniform and reproducible quantities of drugs must be coated onto microprojections. Therefore, optimisation of coating process and formulation characteristics is of paramount importance during developmental stage of the device. The micron-scale dip-coating process was proposed by Gill and Prausnitz (2007a, b) and was successfully applied to the deposition of variety of molecules differing in physicochemical properties on the surface of MNs. The two most important parameters affecting dip-coating process are surface tension and viscosity of the formulation. The authors demonstrated that reduction of surface tension by addition of surfactants (e.g. Lutrol® F-68) and increase in viscosity of the coating solution by addition of viscosity enhancers (e.g. carboxymethylcellulose sodium salt, sucrose) resulted in uniform and thick drug coatings on MNs. It was concluded that coating solutions with lower surface tension facilitated good wetting and decreased speed of film formation on the MN surface. In contrast, higher viscosity (and reduced contact angle of the coating solution) caused increased volume of liquid film adhering to the MNs and increased residence time of the film on the MN surface. The versatility of this coating technique was demonstrated by coating hydrophobic molecule, curcumin, as well as model proteins, BSA and insulin, in either organic or aqueous-based coating solutions onto the surface of MNs. In addition to coating drugs on solid shafts of MNs, the investigators proposed a novel approach where drug could be deposited within holes created in the centre of the MNs (Fig. 18.4) (Gill and Prausnitz 2007b; Kim et al. 2012). In the most complex design, these holes, called ‘pockets’, could be selectively filled with one drug formulation, and, additionally, another protective layer could be coated onto the surface of the MN shaft, followed by the deposition of another drug layer. The authors demonstrated filling the pockets with small molecules such as sulforhodamine and coating fluorescein onto the surface of MNs. The two drugs were separated by the thin protective layer composed of biodegradable polymer poly(lactic-co-glycolic acid) (PLGA) (Fig. 18.6).


Fig. 18.6
Coated microneedles of metal and polymer before (a, c, e, g) and after (b, d, f, g) coating using dip-coating methods. The coating should dissolve in the skin’s interstitial fluid upon insertion to release its drug/vaccine payload (Reproduced with permission from Elsevier, Gill and Prausnitz (2007b) and Kim et al. (2012))
Chen et al. (2009) proposed a novel coating technique utilising gas jet to distribute coating solution evenly onto the surface of the individual MN shafts. The microprojection patch was composed of densely packed (~ 20,000 cm2) solid silicon MNs of a height of 30, 60 or 90 μm. Due to inability to coat such small projections in a conventional dip-coating process, the authors developed a new gas jet-drying method. The coating solution (6–8 μl) was applied to the patch, and gas jet (6–8 m/s) at an incident angle of 20° was used to distribute and quickly dry the formulation on the whole patch. The proposed method allowed for uniform coating of variety of compounds (OVA, rhodamine-labelled dextran, ethidium bromide) on short and densely packed microprojections.
To enable more controlled and efficient delivery, coated MNs have been the most extensively studied technique for vaccination with MNs (Kim et al. 2012). The presence of immune-presenting cells makes the skin an attractive site for antigen presentation. Antigens can be introduced into the skin via coated MNs to target Langerhans cells in the epidermis or dendritic cells in the dermis in order to induce more pronounced immune response (Gill and Prausnitz 2007a). The limited amount of drug that can be coated onto MNs does not hinder their application for vaccine delivery as only minute quantities of antigen are necessary to elicit immune response (Banga 2009). In addition, storing vaccines in a dry state coated onto the MNs may preserve their stability to a greater extent, even at room temperature, than storage dissolved in a liquid form in a conventional needle-and-syringe injection product (Gill and Prausnitz 2007a).
Several research groups focused their efforts on investigations of delivery of vaccines using coated MNs. Investigators at the Georgia Institute of Technology and Emory University, Atlanta, assessed the efficiency of solid metal MNs (500 μm) coated with inactivated influenza virus (IIV) to deliver the antigen to the skin. The results showed that as much as 10 μg of viral proteins could be coated onto an array of five needles and 83 % and 90 % of the antigen were deposited in the mouse skin after insertion of MNs for 2 min and 5 min, respectively. This indicated that the vaccine could be effectively coated onto MNs and rapidly delivered to the skin within minutes. The delivery of IIV via MNs induced antibody responses that did not differ statistically from those elicited by intramuscular injection. In addition, vaccination by a single dose of IIV coated onto MNs protected mice against lethal challenge by a high dose of mouse-adapted influenza virus A/PR/8/34 (Zhu et al. 2009). In another study, Gill and Prausnitz in collaboration with colleagues at the Karolinska Institutet, Stockholm, evaluated cutaneous DNA vaccine delivery using coated MNs in mice. MN array composed of five needles of 700 μm height was coated with plasmid encoding hepatitis C virus NS3/4A. It was found that each array was coated with 1.6 μg of DNA. Mice were immunised with 8 μg of DNA using MNs (five arrays) and for comparison with 4 μg of DNA using gene gun. The results revealed that MN-mediated delivery of the antigen induced the generation of cytotoxic T-lymphocytes which was comparable with that observed after antigen delivery using gene gun (Gill et al. 2010).
Researchers at Zosano Pharma (formerly ALZA Corporation) evaluated the performance of Macroflux® microprojection array for intracutaneous delivery of model antigen, ovalbumin (OVA), in hairless guinea pigs. The Macroflux® skin patch (Fig. 18.7) having an area of 1 or 2 cm2 and comprised of titanium 330 μm high MNs (density 190 MNs/cm2) was coated by immersion in a 1, 5 and 20 % solution of OVA. The results showed that by increasing concentration of OVA solution, the amount coated onto microprojections was increased. In addition, skin deposition experiments revealed that the quantity of OVA delivered into the skin increased linearly with time of application. Hairless guinea pig immunisation studies revealed that at all antigen doses, administration of OVA-coated Macroflux® resulted in immune response comparable to that observed after intradermal administration of the same doses of antigen. However, application of 1 μg and 5 μg of OVA via Macroflux® induced 10 and 50 fold increase in anti-OVA titres in comparison to those produced by intramuscular or subcutaneous injections of equivalent doses (Matriano et al. 2002).


Fig. 18.7
Illustration of (a) Macroflux® patch comprising the coated MN array affixed to an adhesive backing and (b) the patch loaded on the disposable retainer ring and the reusable applicator. Reproduced with permission from Elsevier, Cormier et al. (2004))
In the follow-up study, researchers further assessed the efficacy of Macroflux® system. The influence of dose of antigen delivered, depth of antigen delivery and density of MNs on the elicited immune response were investigated. The number of coatings and concentration of OVA in the coating formulation were used to control the amount of OVA loaded onto the MNs. It was found that antibody response was dose dependent. Different depth of OVA delivery was achieved by using MNs varying in height, namely, 225, 400 and 600 μm. The results revealed that MN’s height did not have significant effect on immune response. Similarly, after immunisation with 2 cm2 MN arrays of different density (140/cm2 and 657/cm2), comparable antibody titres were observed (Widera et al. 2006). Apart from vaccine delivery, the capability of Macroflux® technology to deliver desmopressin across the skin was assessed in hairless guinea pig model. It was demonstrated that a dose of 20 μg of desmopressin was delivered from a 2 cm2 MN array (321 MNs/cm2, height 200 μm) within 15 min to hairless guinea pigs, and bioavailability was as high as 85 % (Cormier et al. 2004). Furthermore, Sathyan et al. (2004) assessed safety and pharmacokinetic/pharmacodynamic (PK/PD) profiles of desmopressin administered via Macroflux® patch to human volunteers (18–45 years). Application of MN patch coated with 25 μg of desmopressin for 15 min resulted in a rapid absorption of the drug with a C max of 268 pg/ml obtained by 25 min. The absorbed drug was found to be pharmacologically active as evidenced by the increase in factor VIII levels. The PK/PD characteristics of the delivery of parathyroid hormone 1–34 (PTH(1–34)) coated onto Macroflux® microprojections array have been recently evaluated in human volunteers (40–85 years) by Dadonna et al. (2011). In phase I clinical studies, MN patches coated with 30 μg PTH(1–34) were applied to different sites (the abdomen, upper forearm or thigh) for 30 min in healthy human subjects, and PK evaluation was performed. For comparison the marketed PTH(1–34) product, FORTEO®, was administered by subcutaneous injections. Phase II studies were conducted in postmenopausal women with osteoporosis to determine the patch dose response (20 μg, 30 μg and 40 μg) compared to placebo patch and FORTEO® injection. In phase I studies, it was demonstrated that application of MN patches, irrespective of the site, resulted in rapid PTH(1–34) plasma occurrence with T max 3 times shorter than that observed after administration of FORTEO® (0.14 h and 0.4 h, respectively). In phase II studies, it was shown that MN patch-mediated delivery of PTH(1–34) was dose dependent as a proportional increase in plasma PTH(1–34) AUC was observed with an increase of dose administered.
Chen et al. (2009) investigated the feasibility of transdermal delivery of OVA coated onto very small and densely packed microprojection array (less than 100 μm in height with density ~20,000 MN/cm2). The results showed that the antibody response after immunisation of mice with OVA-coated MN patch was comparable to that observed after immunisation with intramuscular injection.
Apart from solid metal MNs, polymeric MNs were coated with model antigen, and their efficacy in inducing immune response was studied. Han et al. (2009), in order to increase loading capacity of the MNs, fabricated groove-embedded MNs from biocompatible polymer poly-L-lactic acid (PLA). To test the effect of MN type on immunisation, three types of MN arrays (types A, B and C – Fig. 18.6), all comprised of 15 needles of 880 μm height, were coated by dipping in 100 μg/ml OVA formulation and applied for 10 min to the mice skin (Han et al. 2009) (Fig. 18.8).


Fig. 18.8
SEM images of three different types of the PLA microneedles (Reproduced with permission from Elsevier, Han et al. (2009))
The results showed that the degree of immune response was higher after application of type C MNs, followed by type B and type A. This result suggests that groove-embedded MNs (types B and C) allowed for higher drug loadings in comparison to the smooth ones (type A).
18.2.3 Polymeric MNs
In contrast to coated MNs, a concept of polymeric MNs encapsulating pharmaceuticals within their matrix was introduced. Upon contact with the skin’s interstitial fluid, polymeric MNs either dissolve or degrade releasing drug cargo for local or systemic delivery. A plethora of advantages is associated with the use of polymeric MNs. Firstly, in contrast to silicon which is a material with dubious biocompatibility, a wide range of polymers are biocompatible and have long-standing safety records when used in drug delivery systems and medical devices (Park et al. 2005). The use of water-soluble and biodegradable polymers as well as sugars eliminates the risk of leaving biohazardous sharp waste in the skin as well as guarantees safe MN disposal by mechanical destruction or dissolution in a solvent (Park et al. 2005; Prausnitz and Langer 2008). In addition, low cost of polymeric materials and their easy fabrication in micro-moulding processes allow for their mass production. Thus, through its unique properties, importantly the biocompatibility and biodegradability, which have been a major concern in various drug delivery systems, polymeric MNs are gaining huge importance (Donnelly et al. 2012). However, a disadvantage of polymeric MNs is that drug loading can compromise their mechanical strength and fabrication process, and this may influence the stability of incorporated molecules.
To accurately produce MNs out of polymeric materials, a variety of mould-based techniques, such as casting (Perennes et al. 2006) (with water as the usual solvent) (Kim et al. 2012), hot embossing (Han et al. 2009), injection moulding (Sammoura et al. 2007) and investment moulding (Lippmann et al. 2007), have been investigated. Various polymeric materials which have been efficiently fabricated into MNs include poly (vinyl pyrrolidone) (PVP), poly-L-lactic acid (PLA), poly-glycolic acid (PGA) and poly-lactic-co-glycolic acid (PLGA), polycarbonate (PC), carboxymethyl cellulose (CMC) polyvinyl alcohol (PVA) (Lee et al. 2008; Park et al. 2005; Perennes et al. 2006; Sullivan et al. 2008; Aoyagi et al. 2007; Han et al. 2007; Chu et al. 2010) and poly(methyl vinyl ether-co-maleic acid) (Donnelly et al. 2011). Sugars have also been used to fabricate MNs such as galactose, maltose and dextrin (Donnelly et al. 2009b; Kolli and Banga 2008; Miyano et al. 2005). Additional use of vacuum and/or centrifugal force is sometimes required during the filling of the material into the mould cavities and drying state (Kim et al. 2012). Examples of polymeric MNs are presented in Fig. 18.9.


Fig. 18.9
Examples of dissolving MNs made from water-soluble polymers and biodegradable polymers (a–h) (Reproduced with permission from Elsevier, Kim et al. (2012))
Miyano et al. (2005) reported for the first time the production of MNs from natural sugars. Drug-loaded MNs were prepared by heating powdered maltose to 140 °C for 1 h until maltose candy was formed, which was subsequently followed by addition of the drug and uniform mixing. After cooling, the small quantities of this drug-containing maltose candy were cast into MN moulds at 95 °C to form MN arrays. Arrays of 500 μm high MNs containing ascorbate-2-glucoside (5 % w/w), sodium salicylate (10 % w/w) and calcein (10 % w/w) were fabricated by this method. It was denoted that MNs dissolved within a few hours at humidity of more than 50 % but retained their shapes for at least 3 months at 40 % humidity. The insertion of 500 μm high MNs into the forearm of human volunteers was found to be painless, and needles were reported to dissolve within 5 min (Miyano et al. 2005). In the follow-up study, the authors addressed the difficulties in fabrication of maltose MNs, such as low throughput due to high viscosity of maltose candy, and proposed polyethylene glycol (PEG, Mw = 6000 Da) as an alternative material for MN fabrication. MNs fabricated from PEG were successfully inserted into human cultured skin without breakage which indicated their sufficient mechanical strength to form microchannels in the skin (Miyano et al. 2007).
Banga’s research group investigated the ability of maltose MNs to improve transdermal drug transport (Kolli and Banga 2008; Li et al. 2009, 2010). However, the researchers strangely used these MNs as means of skin pretreatment rather than as actual drug-containing devices. Solid maltose MNs (500 μm in height) were fabricated in a micro-moulding process, and MN-mediated delivery of nicardipine hydrochloride (NH) across rat skin was investigated both in vitro and in vivo. The results showed that pretreatment of full-thickness rat skin with MNs led to a fourfold increase in NH permeation in comparison to passive diffusion. Throughout this research, it was also demonstrated that MNs improved NH transport across rat skin in vivo. The peak plasma NH level (Cmax) of 56.45 ng/ml was observed after 7 h in MN-treated rats, whereas in untreated group, detectable NH levels were seen only after 8 h, and Cmax of approximately 16 ng/ml was observed after 24 h [50]. Likewise, Li et al. (2009) investigated the efficacy of maltose MNs in enhancing transport of model protein, human immunoglobulin G (IgG) (Mw ~ 150 kDa), across full-thickness hairless rat skin in vitro. The influence of parameters, such as MN length (200 and 500 μm), number of MNs (27 and 54 needles) and drug concentration (5, 20 and 40 mg/ml), on IgG delivery across the skin was evaluated. It was found that IgG flux was increased approximately tenfold and fourfold with increase in number and length of MNs, respectively. Furthermore, an increase in IgG concentration up to 20 mg/ml also resulted in increased flux, after which (for 40 mg/ml) no significant change in flux was noted. This was attributed to the saturation of the boundary layer relative to the donor solution. In addition to IgG delivery, the authors demonstrated further successful transport of monoclonal antibody across MN-treated hairless rat skin in vitro (Li et al. 2009). In a follow-up study, investigators assessed MN-mediated delivery of IgG in vivo. For comparison purposes, apart from maltose MNs (500 μm in height) commercially available DermaRollerTM (700 μm in height) was used to pretreat the skin. The results revealed that IgG peak plasma levels were observed after 24 h and were of 7.27 ng/ml and 9.33 ng/ml in maltose MNs and DermaRollerTM-treated groups. The greater IgG permeation enhancement observed after DermaRollerTM skin pretreatment was attributed to the creation of bigger microchannels with an average diameter of 83 μm in comparison to 58 μm formed by maltose MNs (Li et al. 2010).
Apart from maltose, the applicability of another carbohydrate material, galactose, for MN fabrication was investigated by Donnelly et al. (2009b). The authors described a number of difficulties associated with the processing and storage of galactose MN arrays. Drug-loaded galactose MNs (280 μm in height) were prepared in micro-moulding process by melting galactose powder at 160 °C and subsequent addition of model drugs: 5-aminolevulinic acid (ALA) and bovine serum albumin (BSA). It was highlighted that high viscosity of molten galactose and its tendency to solidify prevented preparation of more than two MN arrays at a time, which excluded the possibility of an easy scale-up for mass production. Moreover, MN fabrication resulted in substantial losses of incorporated ALA and BSA (40 % and 100 %, respectively). In addition, storage of MN arrays at ambient conditions led to their deformation within 1 h and complete loss of MN shape in 6 h.
The fabrication of polymeric MNs from biodegradable polymers such as PLA, PGA and PLGA and their efficacy in improving delivery of model compounds across the skin were described by Park et al. (2005). However, in this study polymeric MNs were used to pretreat the skin and did not encapsulate drug molecules within their matrix. MNs varying in geometry (bevelled tip, chisel tip, tapered cone) were fabricated from hot polymer melts using a micro-moulding technique. It was reported that pretreatment of human cadaver epidermis with 20 MNs led to an increase in skin permeability to calcein and BSA by two orders of magnitude, whereas when 100 MNs were used, the permeability was increased by almost three orders of magnitude. Following this, the authors evaluated the ability of drug-loaded PLGA MNs to enhance transdermal delivery of model compounds, calcein and BSA, in vitro. To allow for controlled release of compounds, drug molecules were either directly embedded within the PLGA MN matrix (for rapid release) or first encapsulated within CMC or poly-L-lactide microparticles, and then the drug-loaded microparticles were incorporated into MN matrix (for controlled/delayed release). It was reported from this that calcein encapsulated within PLGA matrix was released within 4 h, and calcein encapsulated within CMC microparticles and then within PLGA matrix was released within 4 days, whereas calcein encapsulated within poly-L-lactide microparticles and subsequently within PLGA matrix was released over the period of 2 months. This study highlighted the feasibility of controlled drug release ranging from hours to months using polymeric MNs (Park et al. 2005).
In all of the aforementioned studies, MN fabrication relied on the use of high temperatures to melt either polymer or sugar matrix. Such strategy can preclude successful encapsulation of fragile molecules such as peptides and proteins in MNs as already demonstrated by Donnelly et al. (2009b). Alternative MN fabrication methods that do not involve exposure of molecules to elevated temperatures were proposed. In particular, Sullivan et al. (2008) described fabrication of rapidly dissolving MNs in the room-temperature photopolymerisation (UV lamp at 100 W at wavelength of 300 nm) of liquid monomer, vinyl pyrrolidone. The PVP MNs obtained by this process were shown to dissolve within 1 min after insertion into porcine skin in vitro. To alter the dissolution rate, methacrylic acid (MAA) was copolymerised, which produced stronger MNs with slower dissolution rates. In addition, it was reported that red fluorescent BSA-loaded PVP MNs delivered the drug into the skin as confirmed by fluorescence microscopy. Furthermore, it was demonstrated that incorporation of β-galactosidase into MN matrix did not affect its enzymatic activity. Lee et al. (2008) proposed fabrication of MNs from aqueous blends of CMC and amylopectin (AP) in a micro-moulding process. Aqueous solutions of CMC were first concentrated by heating at 60–70 °C or under vacuum, whereas AP solutions were concentrated by heating 60–70 °C only. Concentrated solutions of both materials were then cast into micromoulds to form MNs and were dried during centrifugation at 37 °C. The authors designed MNs which enabled either bolus or sustained release of model compound, sulforhodamine B. For rapid release drug was selectively encapsulated within MNs, whereas for sustained release, it was incorporated either into both the MNs and the backing layer or into the backing layer only. This study therefore presented a design that encapsulated molecules within MNs that dissolve within the skin for bolus or sustained delivery and leave behind no biohazardous sharp waste. This is being increasingly recognised as important (Shakeel et al. 2011). Lee et al. (2008) also showed that lysozyme loaded into CMC MN matrix retained its structural and functional activity after 2 months storage at room temperature. This indicated that direct incorporation of peptide and protein molecules into a dissolving MN system is possible with appropriate selection of the MN fabrication process as well as suitable choice of the polymeric formulation used for MN production.
Chu et al. (2010), from the same research group, fabricated dissolvable MNs from 20, 30 and 50 % w/w polymer blends containing PVA and PVP in a ratio 3:1. In order to better control localisation of model drug within MNs and to increase drug loading without excessive wastage, the authors proposed two novel MN designs, bubble and pedestal MNs. This proposition was investigated because it is sometimes desirable to encapsulate drugs only in the MN tips because MNs may not insert fully into the skin (Kim et al. 2012). It was demonstrated that by increasing viscosity of polymer solution or by introducing air bubbles at the base of the MN, the model drug, sulforhodamine B, could be localised to the MN tips (Fig. 18.10). Localising the sulforhodamine B to the MN tips was shown to result in increased skin delivery efficiency compared to evenly distributing the dye throughout the entire device.
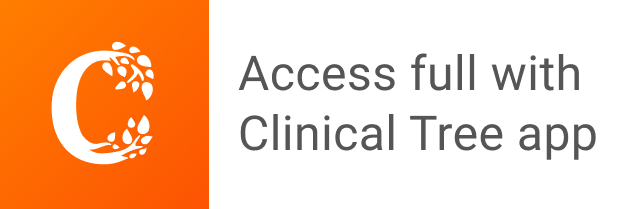