Introduction to Radiation Therapy
Radiation therapy is a treatment modality that involves use of high-energy electromagnetic waves to target and eliminate abnormal cells, and this approach can be used to treat a variety of conditions in the skin and underlying tissues. The clinical effects of ionizing radiation on a cutaneous wound are dependent on the timing of radiation in relation to the process of wound healing. Radiation affects multiple targets in the processes of inflammation, proliferation, and remodeling. These targets make ionizing radiation an effective treatment for prophylaxis of hypertrophic scars and keloids. For lesions that have recurred despite more conservative measures, external beam irradiation using either electron beam or kilovoltage range x-rays may be delivered following local excision. Recurrence rates may be decreased following postoperative irradiation. The dose of radiation delivered to prevent hyperplasia is significantly lower than that used in the treatment of neoplastic processes with relative sparing of deeper critical structures and lower morbidity.
Radiation: Mechanism of Actions, Treatment Modalities, and Definitions
The effects of radiotherapy on human tissue are mediated by the induction of DNA damage and subsequent mitotic cell death. The process begins when incident photons deposit their energy in tissue by ejecting local orbital electrons. These secondary electrons subsequently initiate local ionization events, primarily by reacting with water, producing free radicals, although there is some direct action on cellular DNA. Therapeutic electrons, in contrast to photons, are directly ionizing. The oxidized free radical species produced by ionization induce single or double DNA strand breaks, DNA base alterations or loss, and DNA-DNA and DNA-protein cross-links. The inability of the cell to successfully segregate such damaged chromosomes during mitosis will lead to cell death unless the cell is able to repair the damage.
Treatment is often fractionated, which refers to the delivery of specified radiation doses in temporally separate treatments, and is recommended to both increase the efficacy of effects on target tissue and to allow normal irradiated tissue to repair radiation damage. Thus the schedule of radiation fractionation can be used to both increase efficacy of the dose to the target and minimize radiation damage to normal tissue.
The rationale of fractionated radiotherapy lies in the four Rs of radiation treatment: Redistribution, Repopulation, Reoxygenation, and Repair. Because there is a cell cycle dependence of cellular sensitivity to radiotherapy, temporally separate treatments allow the tumor cell population to redistribute after the sensitive population has been depleted. During the interval between treatments, the cell cycle distribution returns to its baseline, ensuring that a susceptible target cell population will be present prior to the subsequent fraction. Reoxygenation refers to the dependency on oxygen of the oxidization events induced by radiation. There is significant heterogeneity of oxygenation status within a tumor cell population, and the more highly oxygenated cells are selectively killed. Fractionated treatment allows diffusion of oxygen to relatively hypoxic cells, again ensuring a susceptible target population. Differences in the rapidity of DNA repair processes in normal and neoplastic cells account for part of the selectivity of therapeutic radiation for the tumor tissue. The majority of radiation-induced DNA damage is repaired prior to mitosis in all cell types, but because repair in normal tissue is more efficient, subsequent radiation treatment allows for additive DNA damage to be induced selectively in sublethally damaged tumor cells. Repopulation of both normal tissue and tumor cells occurs during a radiation treatment course. The acute side effects of radiotherapy are due to the depletion of normal tissue stem cells, and the repopulation of these is essential to the recovery of normal tissue.
The Systeme International d’Unites unit of radiation dose is the gray (Gy), which is defined as a joule of energy absorbed per kilogram of tissue. An alternate unit of absorbed dose, largely replaced by the gray, is the rad (an acronym for radiation absorbed dose). One gray is equal to 100 rad. Dose is specified to the target volume as defined by the treating radiation oncologist. Epithelial malignancies, for example, are treated to a total dose in the range of 50 to 70 Gy, while hypertrophic scars and keloids are commonly prescribed doses in the range of 4 to 20 Gy.
There are several modalities by which external beam radiation therapy can deliver energy to tissue. The classic form of radiation therapy is photon radiation, or x-ray radiotherapy (XRT), which delivers photon beams produced by a linear accelerator. They interact with matter primarily via electrons in the target tissue, causing secondary ionization via the generation of free electrons. Incident radiation deposits its dose as it passes through matter, becoming attenuated as a function of depth in tissues and the properties of the target. Higher-energy beams, delivered in the megavoltage photon range of 6 to 25 MV, deliver an increased dose at depth in tissue and relatively less at the surface. Lower-energy radiation, such as orthovoltage x-ray, is typically used in the treatment of skin diseases and deposits the dose primarily at the target surface. The most common radiation modalities used to treat skin conditions are:
- 1.
Orthovoltage x-rays refer to photons with maximum energy in the range of 125 to 400 kV. The unit for orthovoltage treatment is not present in all radiation treatment centers. When available, orthovoltage provides the maximal skin surface dosage and is often used in dermatologic applications such as in the treatment of nonmelanoma skin cancer or hidradenitis suppurative and adjunctive treatment of surgically excised keloids.
- 2.
Grenz rays are even lower-energy x-rays in the range of 5 to 15 kV and were historically used to treat superficial benign epidermal conditions such as psoriasis, atopic dermatitis, contact dermatitis, nail dystrophies, and other inflammatory dermatoses. The majority of grenz-ray radiation is deposited at a shallow depth of 1 mm, and deeper structures such as hair follicles and sebaceous and sweat glands are relatively spared. Very few grenz-ray machines are still in use in the United States.
- 3.
Electron beam radiotherapy (EBRT) is a form of external radiation that delivers high-energy radiation, typically in the 4- to 20-megaelectronvolt (MeV) range, using a linear accelerator and is more commonly available than orthovoltage treatment units. EBRT is commonly used in dermatologic applications as they are capable of delivering a high skin dose, and the deposited dose rapidly falls to negligible values at depth in tissue. Electron depth dose, like that of photons, is a function of voltage. Unlike the skin-sparing properties of higher-energy photons, however, increasing the energy of electron beams increases the skin dose. Fig. 6.1 illustrates the dose deposited by various radiation treatment beams, including EBRT, orthovoltage and megavoltage XRT, and grenz-rays, as a function of depth in tissue. Such depth dose curves can be manipulated to optimize dose-to-target versus dose-to-normal structure ( Figs. 6.2 – 6.5 ).
Fig. 6.1
Depth dose curves of various radiation treatment modalities. Therapeutic ionizing radiation deposits energy in tissue as a function of depth. Photon beams deliver their dose at depth in tissue and are relatively skin sparing. Orthovoltage x-rays deliver maximum dose to the skin surface, while further dose deposition is rapidly attenuated.
Fig. 6.2
Dose distribution in tissue varies with energy. Representations of dose distribution in tissue for increasing electron energy ( left column ) and photon energy ( right column ). A single radiation beam incident upon the superior aspect of a tissue-equivalent material ( grey ) will deposit its dose as a function of depth. The lines represent contours of equal dose deposition. For photon energies, after a build-up at shallow depths, dose falls off gradually with depth. For electron energies, this fall-off is more rapid. The total depth shown in each figure is approximately 10 cm.
Fig. 6.3
The use of bolus to alter the depth dose properties of electron beams. (A) demonstrates the depth dose properties of electron beams. Dose deposition rises rapidly, then falls to negligible values. (B) shows how tissue-equivalent bolus material is used to raise the skin surface dose to maximum, while sparing tissue at depth.
Fig. 6.4
The skin-sparing properties of high-energy photons. A comparison of the superficial dose deposition of electron beam ( left column ) versus photon beam ( right column ) radiation. The beam is incident downwards upon the superior aspect of a tissue equivalent material ( grey ). The depth of tissue shown is approximately 3 cm. For electron beams, increasing the beam energy increases surface dose as well as dose at depth. For photon beams, increasing the beam energy decreases surface dose while it increases dose at depth.
Fig. 6.5
The use of bolus to increase surface dose and decrease depth dose. The dose distribution of 6 MeV electrons on tissue equivalent material (A). The dose to superficial tissue is significantly less than 100%, and there is substantial dose deposited several centimeters deep. With the use of bolus (B), the superficial tissue dose is 100%, while deeper tissue is spared.
- 4.
In contrast to the external beam modalities discussed earlier, brachytherapy refers to the application of radioactive sources in close proximity to the target. Brachytherapy can be classified as interstitial or surface, where the applicator is placed within or on top of the target, respectively. Interstitial brachytherapy can be further classified as low-dose rate (LDR) or high-dose rate (HDR). Both LDR and HDR can be delivered using temporary or permanent implant. In temporary implants, the radioactive sources are removed after the desired radiation dose is delivered. In permanent implants, the radioactive seeds are placed permanently in tissues. These seeds decay to background over months to years and remain, inert, in the target tissue thereafter. Interstitial brachytherapy should be avoided in the management of keloid because the trauma caused by the insertion of needles could result in new keloid formation.
Radiotherapy and Patient Selection
Proper patient selection for radiation treatment is especially important in the management of benign disease. The most common concern in treating a healthy young adult with a nonneoplastic proliferative disorder is the incidence of side effects such as wound complications, poor cosmesis, permanent normal tissue dysfunction, and the risk of inducing a secondary malignancy. For these reasons, postexcision radiotherapy is normally considered following more conventional treatments such as excision, intralesional corticosteroid injection, or pressure treatment. Although certain patient populations are prone to posttreatment complications, and certain anatomical subsites are more high risk for long-term side effects, in appropriate patients, low-dose superficial radiation treatment is safe and effective, from both a functional and aesthetic perspective.
Of note, EBRT is specifically limited by its high cost and challenging dosimetry, especially for small or irregular anatomic lesions that may be associated with uncertainties in dose deposition.
Caution should be exercised in the treatment of some patients with nonmalignant systemic disorders that may increase the long-term risks associated with radiation therapy. Several studies have demonstrated that patients with active collagen vascular disease have a several-fold higher risk of late effects, particularly fibrosis. Additionally, some series have implicated radiation treatments in activating systemic flares of such conditions. Notably, all studies demonstrating such sensitivity examined patients treated with higher dose radiation for neoplastic disorders, and there is no equivalent evidence relating to the relatively low doses used to treat benign proliferative processes. Nonetheless, caution in referring and treating such patients should be exercised.
Other systemic diseases that may increase the risk of complications of radiation treatment include hypertension, diabetes, and peripheral vascular disease. All have been shown to increase the risk of late toxicity following irradiation, most notably posttreatment wound-healing difficulties, local infection, fibrosis, and necrosis. Presumably the higher incidence of late complications in these patients is due, at least in part, to the impaired microcirculation caused by the underlying systemic disorder. A radiation-induced effect on locally released cytokines and immune complexes has also been postulated. Again, an increased risk has not been demonstrated in patients treated with low radiation doses.
There are, additionally, some patients with a genetic predisposition to complications or the development of second malignancy following radiation exposure. Defects in DNA damage repair pathways, such as those manifested in Li Fraumeni syndrome, ataxia telangiectasia, or retinoblastoma, do not allow normal tissue to repair radiation-induced DNA damage as effectively, resulting in an increase in posttreatment chromosomal abnormalities and a resultant higher risk of normal tissue damage or second malignancy. Certain congenital immunodeficiencies, such as Bloom syndrome, carry a similar risk due to a similar defect in DNA damage repair. Additional inherited disorders that confer a sensitivity to x-rays are basal cell nevus syndrome (Gorlin-Goltz syndrome), Cockayne syndrome, Down syndrome, Fanconi anemia, Usher syndrome, Nijmegen breakage syndrome, and Gardner syndrome. A careful medical history will clearly identify patients in whom the risks of radiotherapy outweigh any potential benefits.
Particular caution must be exercised in the treatment of children. The same mechanism through which radiotherapy is an effective treatment for disorders of unregulated proliferation can be responsible for long-term developmental abnormalities within the radiation treatment portal. Therapeutic doses of radiation can close bone growth plates and induce organ hypoplasia, making careful treatment planning essential in such patients. These concerns are addressed by the Committee on Radiation Treatment of Benign Disease, which recommends that infants and children only be treated in very exceptional cases and that direct irradiation of certain anatomical areas be avoided. These latter sites include areas prone to high incidence of late effects or increased risk of second malignancy (e.g., breast tissue, thyroid, bone marrow), and those in which the intrinsic sensitivity of such tissue might result in impaired function (e.g., gonads, lens). While the vast majority of such effects described in the literature are in patients treated for childhood neoplasms, late developmental effects of treatment of benign disease have been described, including breast hypoplasia following chest wall irradiation for keloid prophylaxis. This does not necessarily preclude treatment of adolescents or younger children, as the effects of radiation lie predominantly within the treatment field. Consequently, careful treatment planning to eliminate or minimize exposure to noninvolved tissue can minimize the long-term risks in appropriately selected patients.
Radiation Effects on Normal Wound Healing
Ionizing radiation can impair all stages of normal cutaneous wound healing, depending on the anatomic area irradiated, total dose, and timing. Wound healing in previously irradiated skin is impaired due to changes to both parenchymal cells and vasculature, while radiation treatment of healing wounds will have effects that depend on the prior progress through the phases of wound healing. Potential targets of such effects include local inflammatory cells and fibroblasts.
Wound healing in previously irradiated tissue is impaired in a dose-dependent manner. The effects are mediated predominantly through fibroblasts, which have decreased proliferative capacity and permanently impaired function after radiotherapy. There is an attenuation of the normal fibroblast response to growth factor–induced chemotaxis and activation. In addition, tissue is characterized by a decrease in collagen gene expression and failure of complete extracellular maturation. There is some evidence that the latter may be mediated through alterations in growth factor expression, especially transforming growth factor beta. The clinical consequence of this is a significant decrease in wound-bursting strength and an increased risk of wound dehiscence. Studies of wound integrity in irradiated skin demonstrate the most significant compromise in the 3 to 4 weeks following injury. At later time points, the strength of such wounds approaches that of wounds in unirradiated tissue.
The vasculature is also permanently altered by prior radiation. Acutely, there is an increase in vascular permeability, but in the long term there is vascular stasis, occlusion, and edema of vessel walls. This results in poor vascular supply to the irradiated area, which can lead to late dermal fibrosis, resultant loss of elasticity, and a predisposition to infection. Wounds in previously irradiated tissue are slow to heal, prone to dehisce, and more readily require grafting. Grafts are more prone to breakdown, and tissue flaps more likely to fail, especially when the site of origin also lies within the radiation field.
The effects of radiation treatment on existing wounds are primarily timing dependent. Early irradiation can impair the proliferation, migration, and activation of fibroblasts, resulting in decreased collagen formation and cross-linking and decreased wound strength and dehiscence. In contrast, if irradiation is delayed until 3 to 4 weeks after wound formation, there is a much lower likelihood of complication. The probability of such effects seems to be dose dependent, and thus there is a high incidence of such late complications after high-dose radiation, and a lower but significant risk following low doses such as those used in the treatment of benign proliferative processes.
Effects of Radiotherapy on Hypertrophic Scars and Keloids
Radiotherapy is thought to prevent keloid recurrence and induce keloid regression by reversing the histopathologic changes that define hypertrophic scar or keloid formation. In both hypertrophic scars and keloids, there is increased fibroblast proliferation and increased production of type I collagen, and in keloids there is enhanced fibroblast activation in response to growth factors. Studies of the effects of radiation on normal wound healing have demonstrated an inhibition of fibroblast proliferative capacity for treatment administered during the inflammatory phase, consistent with clinical data that suggest optimal efficacy of radiotherapy when it is delivered within 24 to 48 hours of keloid excision. Likewise, irradiated fibroblasts in normal tissue have attenuated collagen production and diminished activation and chemotaxis in response to growth factors. Radiation treatment also induces cell death in some portion of the fibroblast population, diminishing the reservoir of cells available for proliferation and collagen synthesis.
A second postulated mechanism of action of radiation in the prevention of benign proliferative disease relates to monocytes and their sensitivity to radiation-induced programmed cell death (apoptosis). Early in the inflammatory phase of wound healing, a decrease in the population of monocytes would be expected to impair growth factor induction of fibroblast migration, activation, and proliferation. This potential mechanism is also supported by the successful use of radiation treatment in the early postexcision interval ( Fig. 6.6 ).
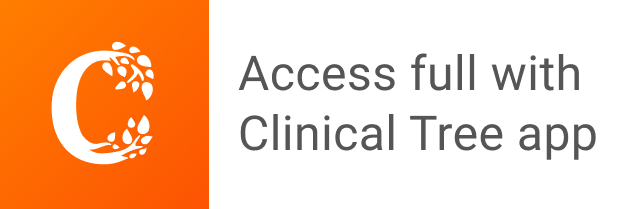