Fig. 14.1
Schematic of liquid jet injector. The components consist of a compartment or cartridge for holding drug formulation, a piston assembly, a power source such as coil spring, and an actuation mechanism

Fig. 14.2
Schematic of drug delivery using liquid jet injector. (a) Formation of liquid jet, (b) initiation of hole formation due to the impact of jet on skin surface, (c) development of hole inside the skin with progress of injection, (d) deposition of drug at the end of hole in a near spherical or hemispherical pattern (spherical pattern shown) (Reprinted with permission from Arora et al. 2008)
Modern jet injectors have clear distinction between disposable and reusable components, with disposable components consisting of parts coming into contact with drug or patient. This makes the drug cartridge and part of piston assembly coming into contact with drug solution disposable. Newer designs have both these components as a part of drug cartridge itself, which minimizes the disassembling task post-injection.
14.2.2 Mechanism of Action
The mechanism of skin penetration and drug deposition in the skin can be divided into two phases, i.e., erosion and dispersion (Baxter and Mitragotri 2005). In the erosion phase, a high-velocity liquid jet impinges on the skin and causes skin fracture due to failure under mechanical stress. This phase is characterized by creation and progression of a hole, formed due to skin erosion along the path of jet progression (Fig. 14.2). During erosion, the jet progresses under submerged conditions and continues to increase the hole depth until it has lost the power required to deepen the hole further. This depth is characterized as the depth where maximum dispersion of drug formulation would occur (Schramm-Baxter et al. 2004). Based on jet power, the drug formulation is deposited in a spherical or part-spherical pattern, with the terminus of the hole acting as a pseudo source of liquid being dispersed. This hypothesis was supported by strong correlation between the depth of maximum dispersion, measured from skin surface, and hole depth, measured across skin samples with varying mechanical properties and jets created with various design parameters. These design parameters are discussed in the following section.
14.2.3 Design Parameters
The fluid delivery profile of a jet injector can be described by percent completeness of injection, penetration depth, and fluid dispersion pattern inside the skin. The design parameters that can be controlled for tailoring this fluid delivery profile of a jet injector include jet velocity, orifice size, pressure profile, and standoff distance.
The penetration of liquid jet into the skin and the percent of fluid delivered into the skin have been shown to be dependent on orifice diameter and jet velocity. Percent delivery of fluid into human skin has been shown to increase in the velocity range of 80–190 m/s for fixed orifice diameter of 152 μm (Schramm-Baxter et al. 2004; Schramm-Baxter and Mitragotri 2004). It is expected that a minimum threshold velocity would be required to rupture the skin, and no penetration would be observed below this threshold. The dependence of percent delivery on orifice diameter was not as strong as that reported for jet velocity. It has been hypothesized that the change in jet structure at higher orifice sizes may result in decrease of percent delivery. The two parameters of jet velocity and orifice diameter have been combined into a single parameter of jet power (P o ). Jet power is calculated as
where D o is the nozzle diameter, u o is the exit velocity, and ρ is the liquid density. Penetration depth increased from 0.2 mm at a power of 1 W to 2.8 mm at a power of 62.4 W (Schramm-Baxter and Mitragotri 2004). With variation in jet parameters, it is possible to span the full thickness of skin and control the depth where the bulk of drug solution is being delivered. The percent completeness of injection also increased linearly from near zero at a power of 1 W to >90 % at a power of ~30 W, beyond which the delivery remained constant at or above 90 % (Mitragotri 2006).

The standoff distance is the distance between the nozzle of injector and the skin at the time of injection. A linear decrease in hole depth was reported for increase in standoff distance for submerged and unsubmerged injections in polyacrylamide gel models (Schramm-Baxter et al. 2004). Some commercial injector manufacturers have accommodated standoff distance by including spacer rings, which can be placed on the skin at the time of injection (INJEX Pharma AG 2013).
An important design parameter is the pressure during injection. In a typical pressure profile representative of spring-powered injector, the pressure rises from the baseline level to a peak of about 3000–4000 psi in under a millisecond, which indicates the actuation (Schramm and Mitragotri 2002). This pressure level is maintained with some oscillations or exhibits a slight drop for the duration of injection. A sudden drop in pressure marks the end of injection. Modulating the pressure profile to better match the delivery of fluid with the rate of fluid absorption by the skin during injection has been an important area of research. To this effect, researchers have developed newer injector designs, which offer more sophisticated control of the jet velocity and thus pressure profiles, such as two distinct phases of higher and lower pressure to breach the skin and deliver the fluid, respectively (Stachowiak et al. 2009; Taberner et al. 2012). To achieve this control, traditional power sources such as compressed spring have been replaced with piezoelectric or Lorentz-force actuators (Stachowiak et al. 2009; Taberner et al. 2012).
In addition to the parameters described above, jet penetration also depends on the skin’s mechanical properties, with Young’s modulus of the skin being inversely correlated to both hole depth and fraction of liquid delivered. This, however, is not the focus of this section and is not discussed in detail. Readers are referred to the work published by Baxter and co-workers (2005).
14.2.4 Applications
MUNJIs have been used for mass immunization programs for diseases including measles, smallpox, cholera, hepatitis B, influenza, and polio (Mitragotri 2005). DCJIs have been used for delivery of several proteins. Most work on DCJIs has been done on delivery of insulin (Lindmayer et al. 1986; Weller and Linder 1966) and growth hormones (Agerso et al. 2002; Bareille et al. 1997; Dorr et al. 2003; Verhagen et al. 1995), while erythropoietin (Suzuki et al. 1995) and interferon (Brodell and Bredle 1995) have also been delivered. Insulin administration by jet injectors led to a faster delivery into systemic circulation, possibly due to better dispersion at the injection site. More recently, Zogenix received approval for SUMAVEL® DosePro®, its needle-free jet injector system for delivery of sumatriptan, indicated for migraine (Zogenix, Inc., USA 2013). To ensure commercial success, companies are now marketing their devices for both pharmaceutical and cosmetic applications. For example, Injex is currently marketing its needle-free jet injector for the delivery of insulin and local anesthetics as well as for cosmetic applications (INJEX Pharma AG, Germany, 2013). However, the acceptance of jet injectors has been low due to variable reactions at the site of administration (see section “Safety” below).
To counter the challenges faced by traditional jet injectors, a novel pulsed microjet was also developed (Arora et al. 2007). This new approach focuses on minimizing pain and bruising by minimizing injection volumes and depth of penetration. The actuation mechanism is based on a piezoelectric transducer and offers strict control over delivery volumes and injection velocity. The high velocity (>100 m/s) of microjets allowed their penetration into the skin, whereas the small jet diameters (50–100 μm) and extremely small volumes (2–15 nl) limited the penetration depth of these jets inside the skin to approximately 200 μm. The efficacy of this design was confirmed by delivering therapeutic doses of insulin in a rat model.
14.2.5 Safety
The acceptance of conventional jet injectors has been limited due to variable reactions at the administration site. Some reports state no difference in the level of pain compared to that experienced by hypodermic needles (Sarno et al. 2000), but others have reported higher levels of pain (Jackson et al. 2001). Variable reports in local reactions further augmented this fact, with some researchers reporting the absence of local reactions (Resman et al. 1985), while others have reported significantly more reactions including pain, bleeding, and hematomas (Houtzagers et al. 1988). It has been shown that the depth of penetration and percent delivery decrease with increasing Young’s modulus (i.e., mechanical strength) of the skin (Baxter and Mitragotri 2005). Commercial injectors come with very limited choice of settings, and owing to the person-to-person variability in skin’s mechanical properties, variability in patient response may be due to the failure of this “one-size-fits-all” approach of current commercial devices. Future devices such as pulsed microjets or Lorentz-force actuator-based injectors are being designed to address these problems by offering superior control over injection profile.
14.3 Powder Jet Injectors
The term powder injectors or projectile injectors describe injector devices used to deliver vaccines or drugs in dry powder or particulate form (Kendall 2006). Other terms such as biolistic injectors and gene guns have also been commonly used for these injectors, with the latter term used exclusively for deoxyribonucleic acid (DNA) delivery, typically on coated microparticles. Early work on powder injectors demonstrated the delivery of genetic material in plant cells via coated tungsten particles (Klein et al. 1987).
14.3.1 Injector Design and Operation
Basic design of solid jet injectors includes compressed gas as the power source, a drug compartment containing particulate drug formulation, and a nozzle to direct the flow of particles (Kendall et al. 2004a; Mulholland et al. 2004). A schematic of solid jet injector is shown in Fig. 14.3. The drug compartment is closed with diaphragms on either side, which are typically few microns thick. Upon triggering the actuation mechanism, compressed gas from a storage canister expands and pushes against the diaphragms, sequentially rupturing them. The flow of gas carries the drug particles with it. The particles then exit through a nozzle and impinge on the skin (Fig. 14.4). Upon impacting on the skin, particles puncture micron-sized holes into the stratum corneum by virtue of their momentum. Some particles are contained in the stratum corneum while a significant percent reach the viable epidermis for the desired therapeutic effect.



Fig. 14.3
Schematic of powder injector. The components shown include a compartment for holding solid drug formulation, a power source such as compressed gas, separating diaphragms, and an actuation mechanism

Fig. 14.4
Schematic of drug delivery using powder injector. (a) Ejection of particles from the nozzle, (b) impact of particles on skin surface, (c) penetration of particles across the stratum corneum, (d) completion of delivery. Particles which penetrate into the skin are mostly distributed in the stratum corneum and viable epidermis (Reprinted with permission from Arora et al. 2008)
Another design used for studying powder injection mechanisms is light-gas gun, which uses an accelerating piston for imparting desired particle velocity (Crozier and Hume 1957). Upon triggering the actuation mechanism, the piston accelerates and carries the particles with it. A deceleration mechanism forces the piston to slow down and makes the particles leave the surface of piston. The particles are ejected and impact on target tissue surface.
14.3.2 Design Parameters
Key parameters in determining particle delivery across the stratum corneum are impact velocity, particle radius, and particle density. The particles constitute powdered preparation of drugs or vaccines and range between 10 and 20 μm. For DNA vaccination, coated metal particles between 0.5 and 3 μm have been used. A much broader range of particle sizes (0.5–52.6 μm) and densities (1.08–18.2 g/cm3) have been studied for injector development (Kendall 2002; Kendall et al. 2004a). Increase in particle size has been shown to increase delivery in an animal model using PowderJect® injector (Isis Innovation Ltd., Oxford, UK) (Sarphie et al. 1997). Diameter of the treated region is another design parameter and has been reported as up to 4 mm. When combined with the key parameters mentioned previously, this puts a limit of several milligrams on the dose delivered. It was also shown that increase in relative humidity and temperature increased the depth of penetration of gold particles from the stratum corneum to the epidermis using a biolistic injector (Kendall et al. 2004b). Hence, relative humidity and temperature may also need to be monitored or controlled for targeted delivery.
For studying correlations between particle properties and skin penetration, a combined parameter, namely, particle impact parameter, has been defined as ρvr, where ρ, v, and r are particle density, impact velocity, and radius, respectively (Rochelle and Lee 2007; Soliman and Abdallah 2011). Particle impact parameter represents momentum per unit cross-sectional area of the particles. The depth of penetration and fraction of particles penetrating the stratum corneum were found to be directly proportional to this parameter. At a fixed value of particle impact parameter, an increase in particle radius corresponds to a decrease in particle velocity at constant density and resulted in a decrease in penetration depth. For a given set of particle properties, varying gas pressure can control the velocity of particles. Typical range of pressures investigated and used is between 200 and 900 psi. Since keeping particle impact parameter uniform is necessary for targeting specific skin layers, various internal contour designs have been studied for achieving narrow velocity profiles. This has led to the optimization of internal sections of the injector, namely, driver tube and shock tube, through which the carrier gas flows before reaching the nozzle (Kendall 2002; Kendall et al. 2004c). A recent study has revealed a correlation between epidermal cell death and particles delivered per unit area of target tissue, making particle payload another important parameter (Raju et al. 2006).
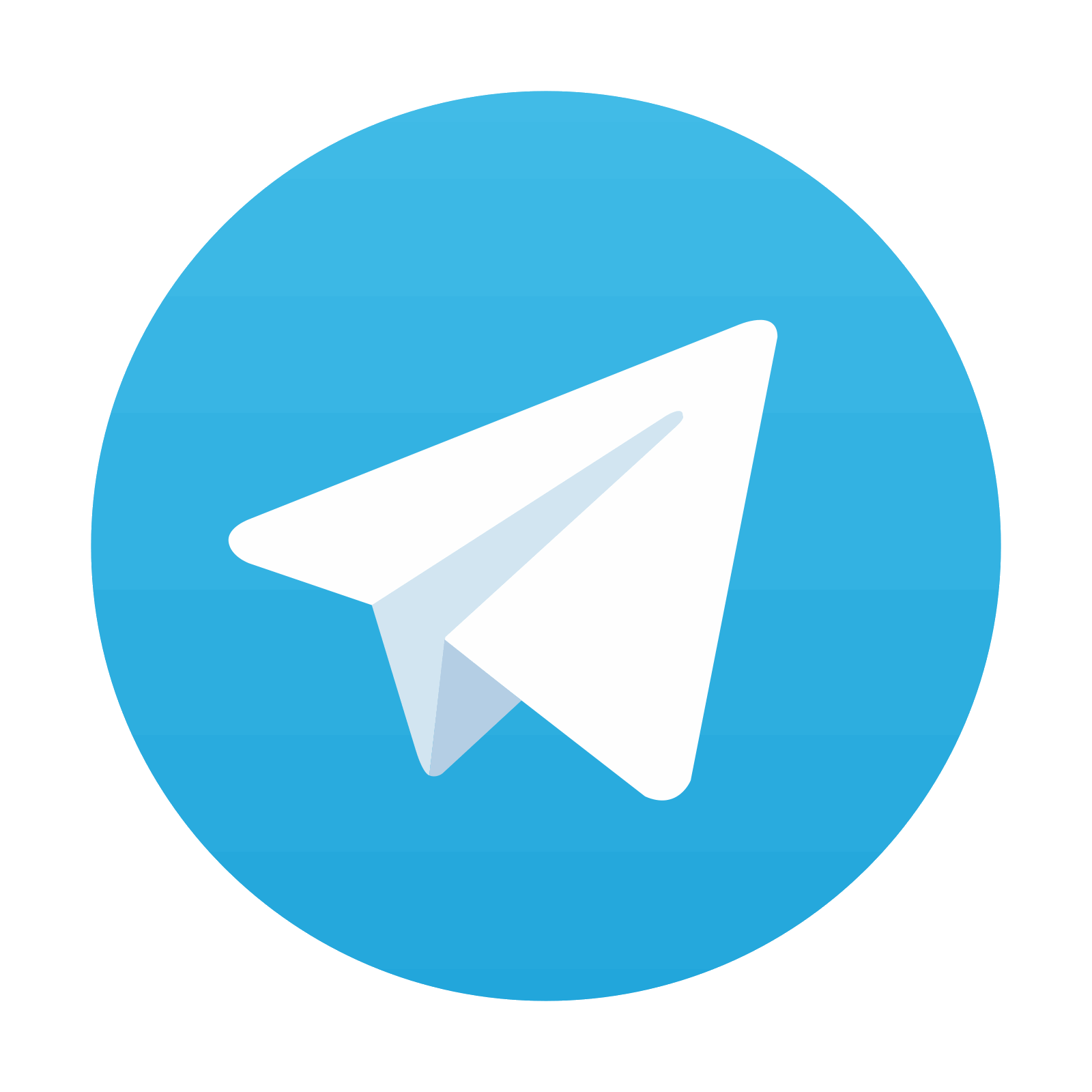
Stay updated, free articles. Join our Telegram channel
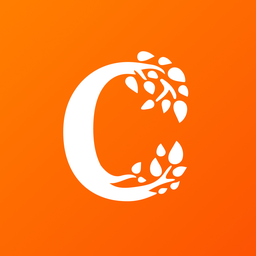
Full access? Get Clinical Tree
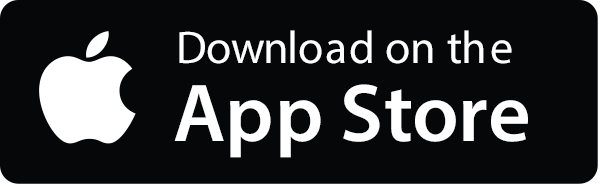
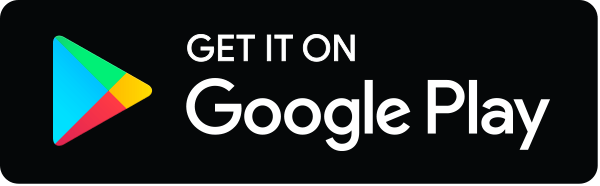