Fig. 29.1
Four-plate electrode. The 4PE is a noninvasive electrode with four plates at perpendicular 90° right angles. A nonconductive stopper maintains a constant gap of 6 mm between electrodes
A later design known as the multielectrode array (MEA) was developed to decrease the sensation of pain by reducing the applied voltage. This was achieved by the addition of electrodes to the design at smaller distances between them. Figure 29.2 demonstrates that the MEA has 16 electrodes with 2 mm electrode spacing (Heller et al. 2010). The reduced distance between electrodes decreases applied voltage by a factor of three while maintaining the electric field (V/cm). For example, using the 4PE an electric field of 300 V/cm would equate to applying an absolute voltage of 180 V (V = EF * (6/10)), whereas that same electric field would equate to an absolute voltage of 60 V (V = EF * (2/10)) using the MEA. Initial publications using the MEA to enhance gene expression demonstrated that luciferase gene expression in guinea pigs and rats was similar to conventional NPEs and the expression was related to the duration and field strength applied (Guo et al. 2011; Ferraro et al. 2011; Donate et al. 2011). Additionally the expression was shown to be maintained within the epidermis and any resultant damage was resolved within 1–2 weeks of treatment (Guo et al. 2011).


Fig. 29.2
Multielectrode array. The multielectrode array is designed with 16 electrodes in a 4 × 4 square array with a 2 mm gap between electrodes. The array allows for application of pulses in two directions. Standard usage of the MEA has been with 72 pulses, pulse ranges of 25–300 V/cm, and pulse durations of 50–300 ms
29.2.2.3 Preclinical Applications
Skin EP has been performed in most animal models including mice, rats, guinea pigs, pigs, rabbits, and rhesus macaques. Experiments evaluating gene expression, wound healing, DNA vaccines, cancer, and systemic expression have been conducted. Studies published prior to 2010 were extensively reviewed by Gothelf et al. (Gothelf and Gehl 2010). Only those publications published since 2010 will be reviewed.
Gene Expression Studies
Electrically mediated gene expression in the skin has been extensively studied with both PE and NPEs. The primary reporters have been β-galactosidase, luciferase, and green fluorescent protein (GFP). To date there is no consensus among researchers as to the “optimal” electrical parameters for gene delivery. Instead the optimal parameters are dependent on a variety of factors including electrode design, field strength, pulse duration, end point assay, etc. Reports using NPEs (in mice and rats) have demonstrated that luciferase expression can be increased 100-fold as compared to skin injection alone at time points between 24 and 48 h (Roos et al. 2009b; Ferraro et al. 2011; Heller et al. 2010). This timing of expression was verified in a 2011 study by Guo et al. where multiple treatments were utilized over time and each time point showed a spike in the expression at 48 h after treatment (Guo et al. 2011). While many publications report peak expression about 48 h after delivery, it is possible that this is an effect of the reporter used for assay, in this case luciferase. A 2010 publication using the Katushka reporter, far-red fluorescent protein, demonstrated peak expression out to day 9 (Gothelf et al. 2010a). The researchers theorized that variation may be related to half-life of the protein. In most cases both PE and NPE have been shown to enhance skin delivery upon application of optimized parameters. However, one publication conducted in porcine skin in 2011 was unable to generate significant increases in luciferase gene expression from plate electrodes in the skin. In that study, significant increases were only seen when penetrating needles with high- and low-voltage pulse combinations were used for delivery (Gothelf et al. 2011).
DNA Vaccines
EP has been used to deliver a variety of antigens including viral, bacterial, and parasitic infections. Success among these antigens has been variable and dependent upon the EP system applied as well as the target organ. Delivery of antigens to the skin with EP to stimulate a response against infectious disease is much more limited. Recent publications have evaluated viral antigens including HBV (Donate et al. 2011), HIV (Brave et al. 2010), influenza (Broderick et al. 2011; Shen et al. 2012; Lin et al. 2012), and smallpox (Hirao et al. 2011). Many skin EP results have demonstrated the induction of a strong lasting humoral immune response. This was evident in the study by Donate et al. where significant increases were seen from weeks 3–24 in a guinea pig model against HBV using the MEA (Donate et al. 2011). Similar results were demonstrated in an electrically mediated smallpox DNA vaccine where an increased humoral immune response was seen with intradermal EP as compared to intramuscular (Hirao et al. 2011). Electrically mediated DNA vaccine studies against influenza have shown promising results as well. Shen et al. achieved 100 % protection in mice from lethal challenge of influenza A after an electrically mediated vaccination (Shen et al. 2012). In a biodistribution study, little DNA was found in the skin and muscle from EP vaccination while still eliciting a strong IFN gamma response against HIV (Brave et al. 2010). Though nonhuman primate studies have resulted in lower immunogenicity responses as compared to small rodent models, protective levels of antibodies have been demonstrated in smallpox and influenza models as well as protection from challenge with monkeypox virus (Hooper et al. 2004; Loudon et al. 2010).
Cancer Vaccines
In the discussion of cancer treatment using EP, there are several different methods that have been employed. The use of irreversible EP (IRE) or tumor ablation has been used to kill tumor cells. While this treatment, depending on electrical parameters, has been successful, it is primarily a localized therapy that is mainly effective against only the IRE-treated tumors. Electrochemotherapy (ECT), chemotherapeutic drugs delivered by electrical pulses, has been used since the early 1990s for the treatment of tumors. The enhancement of drug (such as bleomycin and cisplatin) entry into the cells by the addition of electrical pulses has markedly increased the effectiveness of antineoplastic drugs. ECT has been approved for human use in Europe. Overall response rates in patients with unresectable recurrent tumors were between 80 and 90 %. However, ECT is less effective against deep-seated tumors and metastasis (Escoffre and Rols 2012). Reversible EP has also been used to reduce tumor burden, but instead of destroying the tumor with pulses, genes are delivered to the skin or intratumoral (i.t.) for skin cancers to stimulate the immune system either to recognize tumor antigen or recruit immune cells to the tumor.
Much of the preclinical work for electrically mediated cancer vaccines has been focused on delivery of cytokine DNA for immune stimulation. Upon delivery and expression of these cytokines to the tumor or skin, they are able to stimulate the recruitment of immune cells for tumor recognition. Early reports were published in 2000 and evaluated the combination of ECT with delivery of plasmids encoding either IL-2 or GM-CSF. Their results indicated that long-term immunity could be achieved and upon challenge with new tumor, 25 % of animals were resistant to the new tumors (Heller et al. 2000). These promising results led to several studies which analyzed the use of electrically mediated cytokine DNA without ECT. One such report examined i.t. injection and EP of IL-12 and/or IL-2. A significant delay in the growth of tumors was attained (Lohr et al. 2001). A study by Lucas et al. in 2001 using plasmid IL-12 delivered i.d. by EP resulted in a tenfold increase in IFN-γ levels in serum of treated animals (Heller et al. 2001). Follow-up and concurrent studies by the same group reported that tumor volume could be significantly reduced by treatment EP-delivered plasmid IL-12. Histopathologic analysis revealed that the tissue from EP-delivered plasmid IL-12-treated groups appeared in the best health. No systemic toxicity of IL-12 was noted (Heller et al. 2006; Lucas et al. 2002). A more recent study evaluated the use of plasmid IL-12 with i.t. two-needle EP to reduce tumor burden in beagles with canine transmissible venereal tumor (CTVT). Their results echo those seen from earlier rodent reports demonstrating a reduction in tumor burden and enhancement of immunologic memory to the cancer cells (Chuang et al. 2009). In 2006, Ugen et al. evaluated the effect of IL-15 plasmid delivery by EP (Ugen et al. 2006). Their results showed that IL-15 plasmid induced complete tumor regression and that through the addition of EP, this response could be enhanced. A follow-up study by Marrero et al. using different time points for treatment showed an increase in percent survival as compared to the initial Ugen et al. publication (Marrero et al. 2014; Ugen et al. 2006).
As with the BD devices, tyrosinase vaccines have also been evaluated, but though these are studying melanoma, the EP delivery was performed into the muscle. Results showed a significant delay in implanted tumor growth as well as little to no development of tumor nodules following an intravenous (i.v.) injection of tumor cells (Kalat et al. 2002).
Wound Repair and Metabolic Disorders
Other areas of EP delivery research have included wound repair and metabolic dysregulation. Only a few studies have been performed but their results represent significant findings in these fields. In a 2011 study by Wong et al., a microneedle electrode was used to deliver insulin to diabetic mice. It should be specified that in this study insulin protein was delivered and not the gene. Insulin diffusion increased nearly 100-fold by EP delivery. The addition of heat to the skin for 20 min prior to EP delivery enhanced the diffusion over 200-fold (Wong et al. 2011).
A 2011 study used noninvasive EP delivery in a wound healing model. Ferraro et al. created a random skin flap (RSF) model on the dorsum of the rat. The flap was pulled back from the fascia to sever all blood vessels to that section of the skin and was immediately sutured. After 48 h plasmid DNA expressing vascular endothelial growth factor (VEGF) was delivered by EP at four equally spaced sites on the flap. Perfusion of the flap was measured before and after wounding, as well as over time after EP delivery. Their results showed an increase in VEGF expression for 5 days after treatment and an overall increase in perfusion of the skin flap. The increased perfusion was correlated to an increase in flap survival (Ferraro et al. 2009).
29.2.2.4 Clinical Applications
A 2013 search on www.clinicaltrials.gov using the keyword “electroporation” displays 59 active, recruiting, or completed trials. Of those, eight are being conducted using intradermal EP and an additional seven using intratumor EP of skin-related cancers. However, to date only three have been completed. The first was a study evaluating i.t. injection of melanoma with an EP-delivered plasmid IL-12 followed by EP. Their results demonstrated that EP could be used safely and with little to no adverse effects even when delivering a plasmid cytokine for immune enhancement against melanoma. Additionally, their anticancer effects reversed or stopped tumor progression even treating a limited number of tumors. While 90 % of EP-treated lesions were observed to have a response, two patients were observed to have a complete regression of all treated and untreated lesions. Another seven patients also had response in all treated and some of untreated lesions (Daud et al. 2008). A second study, utilizing plasmid IL-2, has not yet published results on the clinical efficacy of their trial but has reported that there was no toxicity from the treatment (Horton et al. 2008). The final study was to evaluate the use of creams for decreasing pain associated with i.d. EP. To date no results have been published.
29.2.3 Sonoporation (Ultrasound)
29.2.3.1 Introduction and Mechanism
Sonoporation, more commonly called ultrasound (US), involves the use of sound waves for modifying the permeability of cell membranes. Like the other active mechanisms discussed, this allows for the uptake of large molecules like DNA. US is used routinely for imaging muscles, tendons, and internal organs. It is relatively inexpensive and portable as compared to magnetic resonance imaging (MRI) and computed tomography (CT). When used properly it is generally considered safe, posing no known risks to patients. Additionally, it is of interest as a physical noninvasive method for gene delivery due to its wide use and public safety record. Traditional frequencies used for US when imaging are in range of 2–18 MHz. While many frequencies have been evaluated for US-mediated delivery, reports by Tachibana et al. in the early 1990s demonstrated that low-frequency US in the 20–100 kHz range was more effective for transdermal delivery than higher frequencies (Tachibana 1992; Tachibana and Tachibana 1991, 1993).
The mechanism of uptake by US-mediated gene delivery has been attributed to three characteristic effects: acoustic cavitation of microbubbles, radiation pressure, and acoustic microstreaming (O’Brien 2007). Figure 29.3 shows how ultrasound can be used to transfer DNA into cells. The most well-understood US bioeffect is cavitation. Cavitation is the result of a nonthermal interaction between a pressure wave and gas inclusion of aqueous media. It causes mechanical perturbation in the vicinity of active bubbles, which can lead to membrane effects on individual cells. The membrane effects from US vary from the transient opening of holes in the membrane to cell lysis or even fragmentation (Miller and Dou 2004a, b, c). Additionally contrast agents, which are traditionally used in US imaging, have been shown to markedly increase gene expression when used in combination with ultrasound-mediated gene delivery (Akowuah et al. 2005; Rahim et al. 2006a; Duvshani-Eshet et al. 2006; Kodama et al. 2006; Pislaru et al. 2003; Guo et al. 2004; Sakakima et al. 2005). US contrast agents have been designed to aid in the generation of microbubbles and serve as “carrier” molecules for DNA entry. Both inertial cavitation and destruction of microbubbles are capable of producing strong mechanical stress to enhance the permeability of the surrounding tissues. In vitro experiments utilizing US-mediated delivery have demonstrated 1000-fold increases in gene expression (Liang et al. 2004; Michel et al. 2004; Mehier-Humbert et al. 2005; Akowuah et al. 2005; Zhou et al. 2005; Rahim et al. 2006a, b; Fischer et al. 2006; Guo et al. 2006).


Fig. 29.3
Ultrasound and microbubble-mediated gene delivery. Application of ultrasound to gas-filled microbubbles causes destruction of the microbubble leading to an energetic wave which causes permeabilization of nearby cells leading to DNA uptake (Figure reprinted with permission from Elsevier, Liu et al. 2006)
29.2.3.2 Ultrasound in the Skin: Gene Expression and Therapeutics
US has been used fairly extensively in targeting gene delivery to various deep tissues with reporter plasmids (Newman and Bettinger 2007) and therapeutic targets. In addition, US-mediated transdermal drug delivery has been well studied in vivo and shown to be effective at increasing transport. However, skin-related gene delivery has only been directly evaluated in one study. This 2011 preclinical ex vivo breast cancer analysis compared multiple physical methods of delivery including ultrasound. Luciferase-expressing plasmid DNA was injected into the tumor slices. After 90 s a low-intensity ultrasound was applied for 5 min. Gene expression was assessed using the in vivo imaging system (IVIS)-luminescence system 24 h after treatment. In ex vivo samples, US gave the highest gene expression of methods studied (adenovirus, AAV, EP, lipofectin, and direct DNA injection) but did not translate in vivo, due to interference from the blood and skin. They reported that US may be most useful when treating superficial and small nodes of the chest wall (Rajendran et al. 2011).
29.2.4 Plasma Delivery
29.2.4.1 Introduction, Design, and Gene Delivery
Plasma is comprised of a mixture of ionized gases. Plasmas are responsive to electric fields and have high electrical conductivity. High-temperature plasmas (dense plasmas) are associated with lightning, stars, and the sun. In contrast, low-temperature plasmas (low-density plasmas) are associated with the aurora borealis and australis in the northern and southern hemispheres, respectively. The use of plasma as a delivery technique can be considered a “green” technology as it uses energy (electrons) instead of liquid chemicals or heat to accomplish its goals.
Two types of plasmas are known and can be described as thermal and nonthermal. Thermal plasmas denote those where the electrons, ions, and neutral gases have the same temperature and are in equilibrium with their surroundings. Nonthermal plasmas have ions and uncharged molecules that are much cooler than electrons and the carrier gas is therefore only slightly ionized. Due to this, nonthermal plasmas cool down very quickly, within fractions of a second. Nonthermal plasmas are used most commonly in biomedical procedures and are typically utilized at atmospheric pressure.
The most commonly noted biomedical effect of plasma usage is surface decontamination (Morris et al. 2009; Terrier et al. 2009; Scholtz et al. 2007a, b; Kuo 2008). However, in 2005 Ogawa et al. demonstrated that in vitro plasma-mediated DNA transfection could be performed (Ogawa et al. 2005). Later plasma-mediated DNA transfection in vitro was shown to be dependent on DNA concentration and the time of plasma exposure (Sakai et al. 2006). Using in vitro skin and cancer models, a 600 seconds plasma delivery was shown to deliver genes in both keratinocytes and B16F10 mouse melanoma (Connolly et al. 2010).
29.2.4.2 Plasma in the Skin: Gene Expression and Vaccines
The use of plasma-mediated delivery in vivo is in the early stages of preclinical testing. Initial in vivo analysis of luciferase reporter expression in a murine model was conducted by Connolly et al. Mice were injected with 50 μl luciferase plasmid and exposed to a 10 min exposure of either positive or negative helium-based plasma. Plume lengths were 2 cm for negative plasma exposures and 3 cm for positive plasma. Similar gene expression levels were seen from both positive and negative plasma delivery. Both were significantly increased over injection of DNA alone. Additionally they saw no muscle contraction or visual damage to the skin from treatment with their plasma generators (Connolly et al. 2009). Figure 29.4 shows the plume generated from plasma exposure and how this method can be used in gene transfer. In a later study, Connolly et al. investigated the use of plasma delivery to vaccinate against HIV. Various plasma conditions were evaluated, but the most efficient condition resulted in significant increases in antigen-specific antibodies and interferon-gamma spot-forming cells (Connolly et al. 2011, 2012).


Fig. 29.4
Plasma-mediated gene delivery. Plasma was applied to mouse skin to evaluate gene transfer. Image was captured with a 2 min plasma exposure, at 8 kV, and an operating distance of 1 cm (Figure reprinted with permission from the University of South Florida College of Engineering: Mark Jaroszeski, Richard Connolly, and Andrew Hoff)
29.2.5 Magnetofection
29.2.5.1 Introduction and Mechanism
The term “magnetofection” (MF) is defined as nucleic acid delivery under the influence of a magnetic field. MF acts on nucleic acid vectors that are associated with magnetic (nano)particles. Nano-sized magnetic particles are said to be superparamagnetic, meaning they are easily magnetized, but immediately return to an unmagnetized state after removing the field (Furlani and Ng 2008). The magnetic particles when bound to a gene vector and under the influence of a magnetic field can transport the vector to a target tissue, shown in Fig. 29.5. In this way the magnetic field targets uptake to specific tissues (Plank et al. 2003a, b, 2011; Scherer et al. 2002; Plank and Rosenecker 2009; Krotz et al. 2003a, b; Kami et al. 2011). A critical aspect for MF is the assembly of the vector to magnetic particles. Four methods have been described.


Fig. 29.5
Delivery by magnetofection. Magnetic nanoparticles that are associated with gene delivery vectors under the application of magnetic force vectors can be directed toward target cells (Figure reprinted with permission from Elsevier, Plank et al. 2011)
The first is through ligand-ligand interactions. The most common ligand-ligand interaction is streptavidin and biotin technology. The second assembly method is self-assembly by electrostatic or hydrophobic interactions. Via this method the negatively charged phosphate backbone of nucleic acids and the negative electrokinetic (or zeta-) potential of all types of viral particles in aqueous media allow for the assembly with cationic species and particles by electrostatically induced aggregation (Scherer et al. 2002; Singh and Kostarelos 2009). The third method is characterization of magnetic vector formulations, which involves determining hydrodynamic diameter, electrokinetic potential, as well as the stability in the presence of proteins. Finally, covalent coupling of vector and magnetic particles is the most rarely used of the formulation methods (Plank et al. 2011).
29.2.5.2 MF Gene Expression and Preclinical Application
To date only a small number of gene delivery experiments have been performed utilizing magnetofection as a delivery method. A 2003 in vitro study used human umbilical vein endothelial cells delivered by MF to express luciferase and green fluorescent protein. MF delivery increased luciferase expression 360-fold over commercially available transfection reagents (Lipofectamine™ (Life Technologies, Grand Island, NY), FuGENE™ (Promega, Madison, WI), and DMRIE-C™ (Life Technologies, Grand Island, NY)). In addition, transfection efficiency with MF-delivered GFP was 39 %. One limitation was a nearly twofold increase in toxicity (Krotz et al. 2003b). A 2008 in vivo wound healing model was performed in rats. Vascular endothelial growth factor (VEGF) 165 cDNA was delivered to rats by MF in combination with US 7 days prior to making the RSF. Giunta et al. demonstrated that VEGF165 could effectively be delivered by MF in combination with US. A 50 % survival of skin flaps and a significant increase in flap perfusion were seen 7 days postoperative. This effect was hampered without the magnetic field and US treatment (Holzbach et al. 2010).
29.2.6 Abrasion
29.2.6.1 Introduction and Mechanism
As discussed, one of the many important functions of the skin and, more specifically, the stratum corneum is to prevent penetration into the skin. Because of this, removal or disruption of the stratum corneum is a potential method for aiding the delivery of genes into the skin. Examples of methods for disrupting the SC include rough surfaces, tape stripping, and microdermabrasion. Rough surfaces like abrasive pads or sandpaper can be used by rubbing the skin to remove dead cells. Tape stripping is performed by repeatedly placing peeling tape from the skin. Microdermabrasion uses exfoliating media containing crystals or diamond flakes and a suction device to remove the skin cells.
29.2.6.2 Preclinical and Clinical Applications
The most common abrasion technique is the use of abrasive paper. Administration of Bacillus anthracis and influenza vaccines has been done by this method (Matyas et al. 2004; Glenn et al. 2009). A handheld clinical strip-pull device called the Skin Prep System (SPS) was designed by Intercell AG (Austria) and is currently used with many of their transcutaneous vaccines. This device is designed to painlessly remove about 25 % of the stratum corneum. It can be used in conjunction with Intercell AG’s vaccine delivery patch (VDP) and their vaccine enhancement patch (VEP) which contains E. coli labile toxin as an adjuvant. This device has been used against influenza. Hemagglutinin inhibition assays from this study reported moderate improvement over vaccine recipients alone (Glenn et al. 2009). Since then Intercell AG has expanded its research to include recombinant protein and inactivated viral vaccines for Japanese encephalitis, traveler’s diarrhea, and pseudomonas (Tauber et al. 2008; Seid et al. 2012).
Microdermabrasion is a commonly used method for skin rejuvenation but has also been effectively used for vaccination against Vaccinia virus. In this study, full-thickness stratum corneum was able to be removed without further damage to the skin layers and an enhancement in skin permeability to drugs was observed (Kim et al. 2009, 2011; Gill et al. 2009).
29.2.7 Ablation
29.2.7.1 Introduction and Mechanisms
Another method of delivery is ablation. Ablation can be performed by either thermal or nonthermal means. Thermal ablation involves the use of heat to destroy and remove the tissue. This includes radiofrequency ablation (RFA), microwave hyperthermia, high-intensity focused ultrasound (HIFU), IRE, and laser ablation. The induction of heat by the given source is used to destroy the focused tissue, and in most preclinical applications, the target tissue is tumor.
Nonthermal ablation is performed either by the use of high-frequency nonthermal irreversible EP (NtIRE) or nanosecond pulsed electric fields (nsPEFs) and is induced by changes to either the plasma (NtIRE) or nuclear (nsPEF) membranes. NtIRE induces cellular defects to the plasma membrane that the cell is unable to recover and results in cell death. nsPEF induced changes which affect the nuclear membrane inducing apoptosis (Beebe et al. 2003).
29.2.7.2 Preclinical Applications to the Skin
Thermal Ablation
Only a few studies have evaluated gene delivery by thermal ablation methods. One study in 2006, published by Birchall et al., demonstrated that radiofrequency ablation (RFA) could be used to deliver a reporter gene. Human breast tissue was obtained by mastectomy or breast reduction. The ViaDerm™ device (Syneron Medical Ltd., Israel) was used at 700 μs bursts and applied voltages of 290 or 330 V and 100 kHz frequencies. ViaDerm™ was demonstrated to induce microchannels large enough and deep enough to transport 100 nm microparticles. Gene expression studies revealed that β-galactosidase could be delivered and expressed using delivery with this device (Birchall et al. 2006).
In another study, IRE was used to deliver GFP to pig liver. While this study was not done to the skin, it could easily be translated to a skin model for ablation. Conditions used for IRE were 1500 V/cm and 90 pulses for 100 μs pulse lengths. While no quantitative expression was shown in this study, all of the animals expressed GFP when delivery was performed with IRE (Au et al. 2011).
Nonthermal Ablation
The first studies in 2002 by Beebe et al. demonstrated that nsPEF could induce reduced tumor growth by apoptosis (Beebe et al. 2003). An in vivo study by Schoenbach in 2006 demonstrated that melanoma tumors could be reduced by nsPEFs and that their blood flow was reduced all with minimal changes in temperature of 3 °C or less (Nuccitelli et al. 2006). Since then, a 2010 study by Nucitelli et al. evaluated the use of nsPEF to reduce the size of melanoma tumors in mouse skin. They reported the elimination of 17 tumors in four mice using nsPEF conditions that maintained skin temperature below 40 °C (Nuccitelli et al. 2010). In addition they demonstrated that little to no scarring was generated by their treatment further demonstrating the specificity of this method. A study in 2012 evaluated the use of nsPEF against cutaneous papilloma and squamous cell carcinoma. In this study, both in vitro and in vivo analyses were performed to determine pulse length, duration, and number. They reported elimination of primary tumors with one treatment and secondary tumors after a second treatment (Yin et al. 2012).
Delivery of small interfering ribonucleic acids (siRNAs) using nsPEF is also being studied. An initial study by Breton et al. used a single 10 ns pulse applied to giant unilamellar vesicles at various fields from 2 to 5.8 kV/mm. FITC-labeled siRNAs were included to evaluate uptake at the different fields. It was determined that increased uptake occurred with increasing fields (Breton et al. 2012).
29.3 Conclusions and Future Prospects
Noninvasive or minimally invasive techniques are of importance for preventing pain which can be associated with more invasiveness procedures. In this chapter we have discussed a variety of active physical delivery methods and the publications to date which have been performed for skin gene delivery using these techniques. The literature published utilizing biolistic and EP-mediated delivery has been focused on DNA vaccines against infectious disease and cancer. However, in the case of EP, wound models and diabetes are also being performed. Established techniques like US, which are typically used for imaging, are being used to deliver genes and may prove to be a mediator for cancer gene delivery. Newer techniques like plasma and magnetofection are being used in DNA vaccines and to treat wounds. A seemingly simple technique of abrading the skin has been used to deliver DNA vaccines against a variety of infectious agents. Finally, ablation, which was typically used to kill cells, is now being shown to have potential for gene delivery under certain conditions.
While much can be done utilizing these physical methods, overall little is known about the mechanisms by which they act. Future work should include further study into the mechanisms of physical delivery devices to assure public safety and efficacy. Understanding more about the mechanisms by which these enhancers function may shed some light on whether certain techniques are more or less useful for various disease states.
References
Abe A, Furumoto H, Yoshida K, Kato T, Saijo Y, Irahara M (2011) Gene gun-mediated skin transfection with FL gene suppresses the growth of murine fibrosarcoma. J Med Invest 58(1–2):39–45PubMed
Akowuah EF, Gray C, Lawrie A, Sheridan PJ, Su CH, Bettinger T, Brisken AF, Gunn J, Crossman DC, Francis SE, Baker AH, Newman CM (2005) Ultrasound-mediated delivery of TIMP-3 plasmid DNA into saphenous vein leads to increased lumen size in a porcine interposition graft model. Gene Ther 12(14):1154–1157. doi:10.1038/sj.gt.3302498 PubMed
Albrecht MT, Eyles JE, Baillie LW, Keane-Myers AM (2012) Immunogenicity and efficacy of an anthrax/plague DNA fusion vaccine in a mouse model. FEMS Immunol Med Microbiol 65(3):505–509. doi:10.1111/j.1574-695X.2012.00974.x PubMed
Au JT, Wong J, Mittra A, Carpenter S, Haddad D, Carson J, Jayaraman S, Monette S, Solomon SB, Ezell P, Fong Y (2011) Irreversible electroporation is a surgical ablation technique that enhances gene transfer. Surgery 150(3):474–479. doi:10.1016/j.surg.2011.07.007 PubMedPubMedCentral
Beebe SJ, Fox PM, Rec LJ, Willis EL, Schoenbach KH (2003) Nanosecond, high-intensity pulsed electric fields induce apoptosis in human cells. FASEB J 17(11):1493–1495. doi:10.1096/fj.02-0859fje PubMed
Bergman PJ, McKnight J, Novosad A, Charney S, Farrelly J, Craft D, Wulderk M, Jeffers Y, Sadelain M, Hohenhaus AE, Segal N, Gregor P, Engelhorn M, Riviere I, Houghton AN, Wolchok JD (2003) Long-term survival of dogs with advanced malignant melanoma after DNA vaccination with xenogeneic human tyrosinase: a phase I trial. Clin Cancer Res 9(4):1284–1290PubMed
Birchall J, Coulman S, Anstey A, Gateley C, Sweetland H, Gershonowitz A, Neville L, Levin G (2006) Cutaneous gene expression of plasmid DNA in excised human skin following delivery via microchannels created by radio frequency ablation. Int J Pharm 312(1–2):15–23. doi:10.1016/j.ijpharm.2005.12.036 PubMed
Boudreau EF, Josleyn M, Ullman D, Fisher D, Dalrymple L, Sellers-Myers K, Loudon P, Rusnak J, Rivard R, Schmaljohn C, Hooper JW (2012) A Phase 1 clinical trial of Hantaan virus and Puumala virus M-segment DNA vaccines for hemorrhagic fever with renal syndrome. Vaccine 30(11):1951–1958. doi:10.1016/j.vaccine.2012.01.024 PubMed
Braun RP, Babiuk LA, Loehr BI, van Drunen Littel-van den H (1999) Particle-mediated DNA immunization of cattle confers long-lasting immunity against bovine herpes virus-1. Virology 265(1):46–56. doi:10.1006/viro.1999.0032 PubMed
Braun RP, Dong L, Jerome S, Herber R, Roberts LK, Payne LG (2008) Multi-antigenic DNA immunization using herpes simplex virus type 2 genomic fragments. Hum Vaccin 4(1):36–43PubMed
Brave A, Gudmundsdotter L, Sandstrom E, Haller BK, Hallengard D, Maltais AK, King AD, Stout RR, Blomberg P, Hoglund U, Hejdeman B, Biberfeld G, Wahren B (2010) Biodistribution, persistence and lack of integration of a multigene HIV vaccine delivered by needle-free intradermal injection and electroporation. Vaccine 28(51):8203–8209. doi:10.1016/j.vaccine.2010.08.108 PubMed
Breton M, Delemotte L, Silve A, Mir LM, Tarek M (2012) Transport of siRNA through lipid membranes driven by nanosecond electric pulses: an experimental and computational study. J Am Chem Soc 134(34):13938–13941. doi:10.1021/ja3052365 PubMed
Broderick KE, Shen X, Soderholm J, Lin F, McCoy J, Khan AS, Yan J, Morrow MP, Patel A, Kobinger GP, Kemmerrer S, Weiner DB, Sardesai NY (2011) Prototype development and preclinical immunogenicity analysis of a novel minimally invasive electroporation device. Gene Ther 18(3):258–265. doi:10.1038/gt.2010.137 PubMed
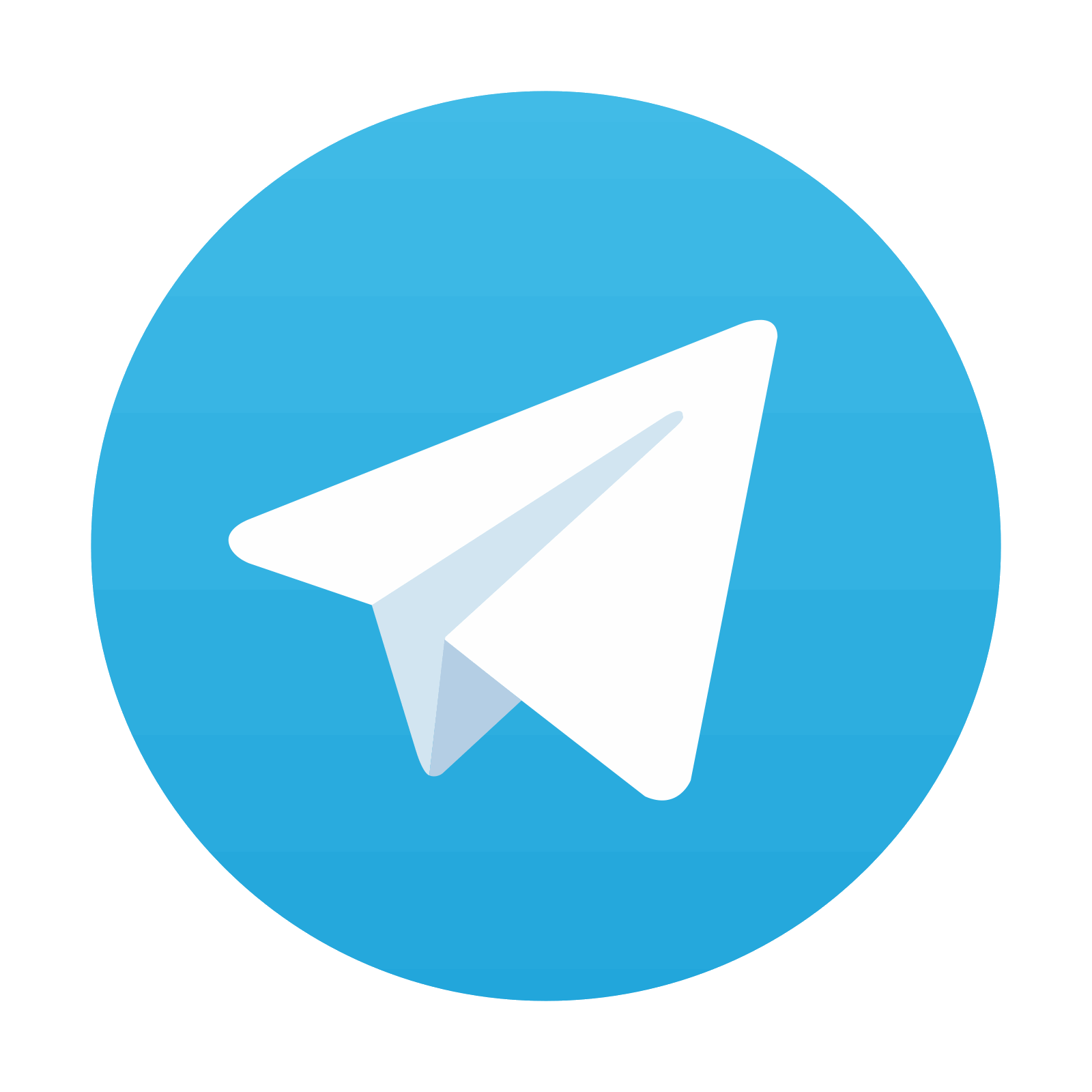
Stay updated, free articles. Join our Telegram channel
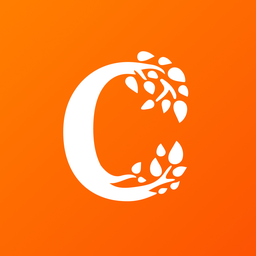
Full access? Get Clinical Tree
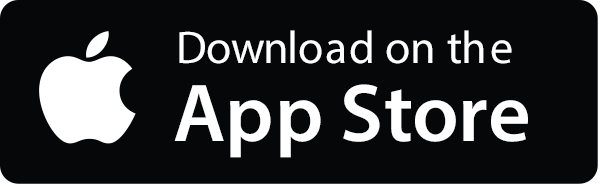
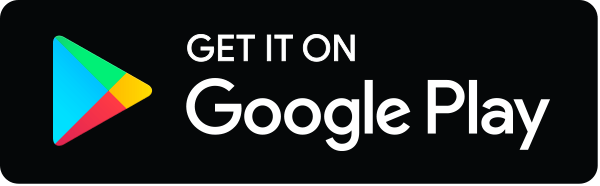