Physical vapor deposition (PVD) techniques
Chemical vapor deposition (CVD) techniques
Others deposition techniques
Thermal evaporation
Plasma-enhanced CVD (PECVD)
Epitaxy
Sputtering
Atmospheric pressure CVD
Casting
Molecule beam epitaxy
Low pressure CVD (LPCVD)
Electrochemical deposition
Ion plating
Very low pressure CVD (VLPCVD)
Silk-screen printing
Laser ablation deposition
Metalorganic CVD
Plasma spraying
Cluster beam deposition
Spray pyrolysis
Casting
19.2.2 Photolithography
Photolithography is a technique used to transfer copies of a master pattern onto the surface of a substrate of some material (usually a silicon wafer). The substrate is covered with a thin film of some material, e.g. silicon dioxide (SiO2) (Fig. 19.1a), on which a pattern of holes will be formed, as seen in Fig. 19.1. There are different types of lithography such as photolithography, electron beam lithography, ion beam lithography or X-ray lithography. Diamond patterning is also an option for lithography. A thin layer of an organic polymer known as photosensitive or photoresist, which is sensitive to ultraviolet radiation (UV), is then deposited on the oxide layer (Fig. 19.1b). A photomask, consisting of a glass plate (transparent) coated with a chromium pattern (opaque), is then placed in contact with the photoresist-coated surface (Madou 1997). The wafer is exposed to illumination; the simplest form is the use of UV, transferring the pattern of the mask to the photoresist, which is then developed (Fig. 19.1c). The radiation causes a chemical reaction in the exposed areas of the photoresist of which there are two types: positive and negative. During the development processes, the rinsing solution removes either the exposed areas or the unexposed areas of photoresist layer, by either wet-etch (using solvent) or dry-etch (using vapour phase or plasma) technique, thereby leaving a pattern of bare and photoresist-coated oxides on the wafer surface (Fig. 19.1d). The oxide layer is etched (Fig. 19.1e). After that, the unwanted photoresist left behind the development process is removed by oxygen plasma treatment (Banks 2006; Donnelly et al. 2012; Madou 1997). The final oxide pattern is then either a positive or negative copy of the photomask pattern and used as a mask in subsequent processing steps (Fig. 19.1f). In MEMS, the oxide is used as a subsequent mask for either further additional chemical etching creating deeper 3D holes or new layers on which to build further layers, resulting in an overall 3D structure or device (Banks 2006; Madou 1997).


Fig. 19.1
Sequential processes in the transfer of a pattern to the substrate surface. (a) the substrate is covered with a thin film of SiO2. (b) a thin layer of photoresist (Photosensitive) is deposited onto the layer of SiO2. (c) a photomask is placed onto the photoresist layer. The wafer is exposed to e.g. UV and the pattern of the mask is transferred to the photoresist. (d) the unexposed area of the photoresist layer is removed and a pattern of bare and photoresist-coated oxide layer is left on the wafer surface. (e) the oxyde layer is etched. (f) the unwanted photoresist left is also removed and the final oxide pattern is obtained.
19.2.3 Etching
Etching is a technique to cut the unprotected parts of a material’s surface by using strong acid or physical process to create a design in it and can be divided into two categories: wet etching or dry etching. The selection of any of the abovementioned methods largely depend on the material of construction and the type of MN (Donnelly et al. 2012). It is used to etch the thin films previously deposited and/or the substrate itself. In wet etching the material is removed by immersion of a material (typically a silicon wafer) in a liquid bath of a chemical etchant. These etchants are classified into isotropic or anisotropic. Isotropic etchants attack the material at the same rate in all directions. On the other side, anisotropic etchants etch material at different rates in different directions, so it is faster in a preferred direction. Potassium hydroxide (KOH) and tetramethyl ammonium hydroxide (TMAH) are the most common anisotropic etchants. Structures formed in the substrate are dependent on the crystal orientation of the substrate or wafer. The dry-etching technology can be divided into three classes called reactive ion etching (RIE), sputter etching and vapour phase etching. Deep reactive-ion etching (DRIE) is a higher-aspect-ratio, up to 50:1, etching method that involves an alternating process of high-density plasma etching in combination with CVD. This process, called BOSCH, was patented by Laermer and Schilp at Robert Bosch GmbH in 1994 (Laermer and Schilp 1996). For silicon, DRIE is one of the most important promising technologies for high volume production. Although the BOSCH process provides a tool to optimise fabrication parameters to achieve high etch rate, high aspect ratio, straight sidewalls and small sidewall scalloping. The probability of getting non-vertical, tapered sidewalls is limited (Roxhed et al. 2007).
19.3 Microfabrication of MNs
MNs are classified as in-plane, out-of-plane or a combination of both, as seen in Fig. 19.2, based on MN design. Considering the in-plane designs (Fig. 19.2a), the MNs are parallel with the machined surface of the substrate (e.g. Si wafer); the major advantage of in-plane MNs can be easily and accurately controlled by the production of the MNs with various lengths during fabrication process. Whereas in out-of-plane designs (Fig. 19.2b), the MNs are perpendicular to the fabrication surface of Si wafer, and it is easier to produce in arrays than in-plane (Ashraf et al. 2011; Donnelly et al. 2012).
The shape and geometry of MNs are critical during design and fabrication. The needles must be capable of inserting into the skin without breaking, and the needles should be of suitable length, width and shape to avoid nerve contact (McAllister et al. 2003; Park et al. 2005; Yung et al. 2012). The elastic properties of human skin can prevent MN from penetration by twisting around the needles during MNs application, particularly in the case of blunt and short MNs (McAllister et al. 2003). Metals are typically strong enough, whereas MNs fabricated from polymers should have sufficient mechanical strength. Typical MN geometries vary from 25 to 2500 μm in length, 50 to 250 μm in base width and 1 to 25 μm in tip diameter (McAllister et al. 2003; Yung et al. 2012).
MN can be classified as solid, coated, dissolving, or hollow according to the structure. MN can also be classified on the basis of overall shape and tip, ranging from cylindrical, rectangular, pyramidal, conical octagonal to quadrangular, with different needle lengths and widths. The tip shape of MN is important for skin penetration, because sharper MNs have higher potential for penetrating the skin, but larger tip diameters require higher insertion forces, which may lead to bending or breaking of the needles in the skin (Arora et al. 2008; Banga 2009; Teo et al 2006). In addition, the shape of the tip of a hollow MN is essential for the flow rate, the flow from a blunt-tip MN supports lower than a bevel-tip MN, because a blunt-tip MN compacts the skin and thus has higher risk of clogging (Bodhale et al. 2010; Luttge et al. 2007). To overcome this problem, it should have a very sharp tip with the bore of the MN off centred or on the side of the MN. Increasing the number of bores in a hollow MN will increase the flow rate; nevertheless, this results in decreased strength of MN and a reduction in sharpness (Stoeber and Liepmann 2002). Figure 19.3 summarises the multiple geometries of MN available.


Fig. 19.3
Shapes of MNs: (a) cylindrical, (b) tapered tip, (c) canonical, (d) square base, (e) pentagonal-base canonical tip, (f) side-open single lumen, (g) double lumen, (h) side-open double lumen (Adapted from Ashraf et al. 2011)
19.3.1 Design and Fabrication of Solid/Hollow MNs
As the name suggests, these MNs are either a solid, with no openings in the structure, or a hollow with a bore/opening within the needle. In order to utilise these MNs for drug delivery, a variety of materials have been used for manufacture, such as metals (Gill and Prausnitz 2007; Matriano et al. 2002), silicon (Gardeniers et al. 2003), glass (McAllister et al. 2003), nonbiodegradable polymers (Jin et al. 2009; Moon et al. 2005) and biodegradable polymers (Park et al. 2005). The most common materials are discussed below.
19.3.1.1 Silicon-Based Solid/Hollow MNs
Prausitz’s research group from Georgia Institute of Technology, Atlanta, USA fabricated the first MN which was made up of silicon. The Si wafer is etched by an oxygen/fluorine plasma mixture in a RIE (a dry-etching process) with a chromium mask. The dimensions of needles were approximately 80 μm at the base, 150 μm in length and approximately 1 μm radius of curvature at the tip. These needles were capable to increase skin permeability of calcein, insulin and bovine serum albumin (Henry et al. 1998; McAllister et al. 2000). Wilke et al. (2005) fabricated silicon MN by a dry-etching technique (a modified RIE). Using a standard wafer of 525 μm thickness, conical-shaped solid MN with an aspect ratio of 4.5:1 (height: base diameter). As usual, undercut etch rate to vertical etch using sulphur hexafluoride/oxygen (SF6/O2) was utilised with the BOSCH-DRIE process (Wilke et al. 2005).
Another type of solid silicon MN called micro-enhancer arrays was etched from silicon wafers using lithography and potassium hydroxide etching, and it was able to deliver naked plasmid DNA into mice skin. These needles measure 50–200 μm in length over a 1 cm2 area and have a blunt tip (Mikszta et al. 2006). This study showed the feasibility of using blunt-tipped MN to scrape the skin for the increased delivery of DNA vaccine to generate an immune response using MN.
Roxhed et al. (2008a) fabricated a sharp hollow silicon MN tips with side openings. In addition, the tips were sealed with a layer of gold coating to yield a closed-package system. The MN was made on a 600 μm thick, monocrystalline silicon wafers using a two-mask process, an anisotropic DRIE etch through the BOSCH process and an isotropic SF6 plasma etching. There were two designs of MN: a 310 μm long cross-shaped and 400 μm long circular-shaped. Three different methods to open the gold seals were used: namely, burst opening, opening upon insertion into the skin and electrochemical opening (Roxhed et al. 2008a). Moreover, Roxhed et al. (2008b) integrated the 400 μm long, circular-shaped HMN (hollow MN) with electrically controlled liquid-dispensing unit to form a patch-like drug delivery system. This liquid dispenser made up of three different layers: a 500 μm thick printed circuit board (PCB) heater layer, a 500 μm thick expandable layer (a mixture of silicone elastomers) and a liquid reservoir (total volume of 12 μL). This integrated device used for delivery of insulin in diabetic rats showed consistent control over blood glucose levels (Roxhed et al. 2008b).
19.3.1.2 Metal-Based Solid MNs
Metal MNs have good mechanical strength, are easy to fabricate and relatively inexpensive, and the metals used, such as stainless steel, titanium and nickel, have established safety records in FDA medical-approved devices (Gill and Prausnitz 2007). They have been fabricated by laser cutting (e.g. stainless steel), wet-etching (e.g. titanium), laser ablation and metal electroplating methods (Kim et al. 2012). The smallest used hypodermic needles (30/31 G) were translated into an array of MN. A research group at Alza Corp. reported titanium MN arrays (commercially called Macroflux®) which were fabricated by applying a thin layer of photoresist onto a titanium or stainless steel sheet, contact-exposing and developing the resist with the desired pattern and bending the arrays to a 90° angle (relative to horizontal sheet plane), which ranged from 175 to 430 μm in length, 190–320 arrays/cm2 over an area of 2 cm2, base width 170 μm and thickness 35 μm. These microprojection arrays were able to deliver oligodeoxynucleotides, ovalbumin, synthetic peptide and human growth hormone across hairless guinea pigs’ skin (Cormier and Daddona 2003).
Omatsu et al. (2010) fabricated MN on a metal surface based on laser ablation using circularly polarised optical vortices having nonzero total angular momentum, known as twisted light with spin, for the first time. The needle showed a height of at least 10 μm above the target surface and a tip diameter of less than 0.5 μm. They also demonstrated the fabrication of a two-dimensional 5 × 6 MN array. This technique forms a metal MN by deposition of a few laser pulses onto a metal target, significantly improving the time and cost of fabrication of two-dimensional metal MN arrays (Omatsu et al. 2010).
Bai et al. 2012 fabricated micro-sized nickel needle array by the Lithographie, Galvanoformung, Abformung (LGIA, eng. Lithography, Electroplating, and Molding) process which is a high aspect ratio fabrication technique based on polydimethylsiloxane (PDMS) mould and nickel transfer technology; the density of array was 900 MN/cm2, and the height was 150 μm. The advantages of this fabrication method are forming complex 3D micro metal structures, low cost, and high throughput.
19.3.1.3 Ceramic-Based Solid Microneedles
The use of ceramic materials raised the possibility to fabricate solid and porous MN, which can be loaded with liquid for drug delivery or diagnostic sampling (Bystrova and Luttge 2011). Solid ceramic MNs were fabricated by micromolding alumina slurry using a PDMS-MN mould and ceramic sintering (Donnelly et al. 2012; Kim et al. 2012). Bystrova and Luttge (2011) from University of Twente, The Netherlands, fabricated ceramic MN by the micromachining of the SU-8 (epoxy photoresist)/Si master, which allows a variety of needles geometries such as disc shape. The multiple replication of the PDMS mould gives a low cost production mould, which can be reused for ceramic MNs fabrication (Bystrova and Luttge 2011).
Ceramic MNs have also been lithographically fabricated using a two-photon-induced polymerisation approach. An intense laser was scanned within a photosensitive polymer–ceramic hybrid resin using a galvano scanner and a micropositioning system to induce polymerisation locally in the shape of the MN (Bystrova and Luttge 2011; Kim et al. 2012).
19.3.1.4 Coated Solid MNs
The micron lengths of needles enforce special coating formulation to obtain uniform coatings and spatial control over the region of the MN to be coated. Because of the effects of surface tension, capillarity and viscous forces become more prominent at these small length scales. Therefore, coating drug formulation onto solid MN should be composed of surfactants to facilitate wetting and spreading of drug solution on the MN surface during the coating process, viscosity enhancers to increase coating thickness and stabilising agent to protect and stabilise biomolecules during drying and storage (Choi et al. 2012; Gill and Prausnitz 2007). In addition, coating solution excipients and solvent should be safe for human use, and the coating method should be compatible with manufacturing processes and not damage-coated drugs.
In 2012, Peters and colleagues from Zosano Pharma, Inc. California, USA, demonstrated for the first time that erythropoietin Alfa (EPO) can be formulated at high concentration and coated onto a MN patch without loss of efficacy or formation of insoluble aggregates. In this study, titanium MN arrays were made by a photo/chemical etching, and the drug formulation which was 15 % w/w EPO, 15 % w/w sucrose and 0.2 % w/w polysorbate 20 coated on the MN array, spins at 50 rpm, in a drug formulation reservoir (2 ml in volume) to produce a thin film of drug with controlled thickness of ~100 μm. The tips of needles are dipped into the thin film and the coating per area adjusted by the number of dips. The time between each dip coating was less than 5 s, which was enough to allow the dryness of the coating. The dose per patch was also optimised by the MN array area (from 0.3 to 3 cm2) (Peters et al. 2012). DeMuth et al. (2010) fabricated multilayer-coated MN, which achieves transcutaneous delivery of plasmid DNA to the epidermis. Plasmid DNA was delivered to the skin by MN application to achieve co-localization with Langerhans dendritic cells (DeMuth et al. 2010)
19.3.1.5 Hollow-Type MNs
Hollow MNs (HMNs) are of interest for pharmaceutical application because they enable transfer of a wide range of molecules transdermally with the advantages of hypodermic injection such as rapid onset action without the drawbacks (e.g. pain, skin reaction). The flow rate can be modulated for a rapid bolus injection, a slow infusion or a time-varying delivery rate. HMNs can be integrated into a smart biomedical device consisting of a biosensor and blood sampling and drug delivery systems (Donnelly et al. 2012; Kim et al. 2012; van der Maaden et al. 2012). HMN arrays are also used as minimally invasive monitoring devices for biological fluid collection and assay (when integrated with other devices).
HMNs were made of glass; polymer and metal have been prepared from substrates by conventional fabrication methods. These needles have been produced either from material substrate of MEMS directly or from multiple substrates with different physicochemical properties to utilise as a sacrificial layer and fabricated by different techniques including laser micromachining, deep reactive ion etching of silicon, an integrated lithographic moulding technique, deep X-ray photolithography and wet chemical etching and microfabrication (Kim et al. 2012).
McAllister et al. (1999) demonstrated the first out-of-plane HMNs. The fabrication was combining solid silicon MNs with the BOSCH process to form a needle bore, 150 μm long HMN and microtubules. McAllister et al. (1999) also presented the fabrication of metal HMN which was fabricated by electroplating the needle (lost-mould technique), had a bore opening of 10 μm in diameter and penetrated epidermal tissue in vitro. Kim et al. (2004) demonstrated later the same way to fabricate metal HMN by electroplating needles on solid MN arrays made of SU-8. The silicon HMN was fabricated by the BOSCH process to create hollow shell structures with high aspect ratio, after which isotropic and wet-etching processes were used to obtain sharper tips. To obtain polymer HMN, the drilling process to make the bore hole and milling to create the bevelled tip shape out of polyphenylsulfone polymer were utilised (Daugimont et al. 2010)
19.4 Design of MNs for Minimally Invasive Monitoring of Biological Samples
Monitoring of analytes in biological fluids represents an important aspect of modern day healthcare. This may be in the form of drug level measurements, as in therapeutic drug monitoring, or could involve the monitoring of key biomarkers for diagnostic purposes or in the management of disease states. Little true progression, however, has been realised within clinical practice with regard to the methods used to extract these analytes, and, for most measurements, conventional blood sampling remains routine, despite the renowned drawbacks associated with this invasive method. For example, hypodermic needles are associated with risk of needle-stick injuries and cross-contamination, such as 37.6 % of hepatitis B infections, 39 % of hepatitis C infections and 4.4 % HIV/AIDS (human immunodeficiency virus infection/acquired immunodeficiency syndrome) infections among world healthcare workers (Rapiti et al. 2005). In addition to the health implications, needle-stick injuries also represent a significant burden economically, with one study estimating an annual cost of £500,000 per NHS (National Health Service) trust (Ball and Pike 2008). Premature neonates, in particular, can exhibit blood volumes as low as 80 ml/kg, making blood sampling to any degree far from ideal and frequent sampling increasing the risk of anaemia (Koren 1997). From the patient’s perspective, minimally invasive sampling offers less discomfort and could offer significant benefits for those with needle phobia and low sample volumes.
Minimally invasive monitoring methods include the use of reverse iontophoresis (Leboulanger et al. 2004; Bouissou et al. 2009; Ching et al. 2011, Ebah et al. 2012), reverse iontophoresis combined with electroporation (Lee et al. 2010), low frequency ultrasound capillary microdialysis (Kim et al. 2008; Nielsen et al. 2009) and by pore creation using a near infrared laser (Venugopal et al. 2008), to name just a few. Interstitial fluid monitoring (ISF) is common practice and an alternative technique to blood extraction (Liu et al. 2005, 2007; Wang et al. 2005; Sun et al. 2010; Ching et al. 2011). While the majority of minimally invasive extraction methods remove ISF, MN has been explored for both blood and ISF sampling, with some claiming an adaption to either matrix possible. For ISF, MN penetration depths of 50–150 μm have been reported satisfactory, whereas arrays designed for blood extraction should offer much larger penetration depths, with values of around 400 μm (Khanna et al. 2008). Others report penetration values as large as 1 mm (Gardeniers et al. 2003) or 1500 μm (Chaudhri et al. 2010), as necessary for successful blood withdrawal. A penetration depth of 325 μm (Mukerjee et al. 2003) is unlikely to guarantee successful blood access; however, considering penetration into the dermis is required for blood capillary targeting, with investigations recording that the epidermis possibly extends as far as 400 μm below the skin (Donnelly et al. 2012). Various different approaches to monitoring using MN have been proposed including the use of HMN arrays for fluid collection and analysis, solid arrays for pretreatment and subsequent fluid collection, as well as integrated options which negate the need for fluid removal entirely. Regardless of the approach, to represent a valuable alternative to current practice, the MN device must be capable of successful and reproducible penetration without fracture and enable accurate measurements of the target analyte.
19.4.1 Considering Fluid Flow in MN Fabrication
Adequate fluid sample is necessary for monitoring, and fluid flow between 1 and 100 μl h−1 has been reported for fluid collection (Gardeniers et al. 2003). Many designs rely on passive extraction alone, depending on capillary action alone, to generate fluid flow. One such design proposed uses a bi-mask process to achieve sharp tips, a cylindrical body and side ports to minimise blockage of the hollow MNs and suggested for use in either drug delivery or microbiological sampling (Zhang and Jullien 2003). The authors recommended that this design is easily fabricated to provide a high needle density and offers a low flow resistance and good structural strength. In a paper that follows, they describe this design further and investigate the performance (Zhang and Jullien 2005). Where the needles, fabricated using silicon dioxide, were capable of human skin penetration without breakage, but passive liquid extraction was only demonstrated in a potato sample. Another early study describing a system amenable to both ISF and whole blood used a HMN array with integrated fluidic microchannels, fabricated using silicon and glass and conducted preliminary tests on the ear lobe of one human volunteer (Mukerjee et al. 2004). The authors outline three needle tip designs including a ‘volcano-like design’, a ‘micro-hypodermic’ design and a ‘snake-fang’ design shown in Fig. 19.4. A common issue with hollow designs is the potential for blockage, and the first two listed here exhibited this issue. The third, ‘snake-fang’, design was thus reported superior since it was found less susceptible to this difficulty, with the bore here placed 25 μm off centre. The fluid capture from the ear lobe by capillary action was described, but penetration testing conducted on the first knuckle of the thumb with the conclusion that a 1.5 N ± 0.25 N force was required for penetration. The risk of glass becoming embedded within the skin represented a major drawback with this design.


Fig. 19.4
Scanning electron micrographs (SEMs) of (a) ‘volcano’ microneedle design, (b) ‘hypodermic’ microneedle design and (c) ‘snake fang’ microneedle design (Reprinted with permission from Elsevier, from Mukerjee et al. 2004)
Other works have focussed more on the theoretical considerations behind fluid flow, driven by capillary action, in hollow MNs and the development of models and calculations to aid in the determination of optimal MN design for optimum fluid flow. Work by one group developed a theoretical fluid model to enable interpretation of microfluidic properties of Newtonian fluids within silicon MN and concluded a faster fluid filling when the length width ratio of the microchannel was √2 + 1 (Liu et al. 2006).
The employment of arrays with a high-needle density has been suggested a possible means to enable sufficient rates of flow, with the use of out-of-plane arrays recommended most appropriate for achieving such specifications (Gardeniers et al. 2003). Contrasting this, others discuss the need for more active extraction methods to ensure adequate fluid collection rather than reliance of capillary force alone. The use of vacuum force to assist with fluid withdrawal has been described (Tsuchiya et al. 2010), as well as more novel approach such as exploitation of the phase transition of a gel to power sample extraction. The latter approach was first documented in the literature using poly(N-isopropylacrylamide) integration within a microsystem intended for glucose sensing (Kobayashi and Suzuki 2001). The volume change exhibited by the gel in response to a variation in temperature was utilised in this study to power a micropump for fluid extraction, with the ultimate aim of achieving spontaneous sampling in response to the temperature of human skin. While successful sampling of a glucose solution was achieved through a 50 μm diameter MN using this concept, flow was only unidirectional and, in response to temperatures, shifts between 30 and 40 °C and, thus, was not relevant for human body temperature. Work to instigate sampling at the more relevant temperatures (30–37 °C) was subsequently carried out (Suzuki et al. 2002). The improved design incorporated a silicon membrane to enable repeated gel use as a result of its elastic force. By shifting the system between hotplates maintained at 30 °C and 37 °C, volume changes were found to occur within less than 1 min, and 90 % response time of the sensor reported to range between 30 and 40 s. This modified system could also successfully achieve bidirectional flow; however, the use of nonideal materials, such as silicon, for MN fabrication is again declared a challenge by the authors. Further work by this group then explored the possibility of continuous monitoring adopting this approach. Adjustment of pH, in addition to temperature, to induce the volume change was used. This study also explored the use of an enzyme-loaded gel to elongate the time for volume reduction even further (Suzuki et al. 2004). Using this combined approach of pH and temperature-induced changes, the time for sampling was prolonged, while the use of the enzyme-loaded gel provided opportunity for further adjustment. The work also demonstrated continuous glucose sampling using an external glucose sample solution.
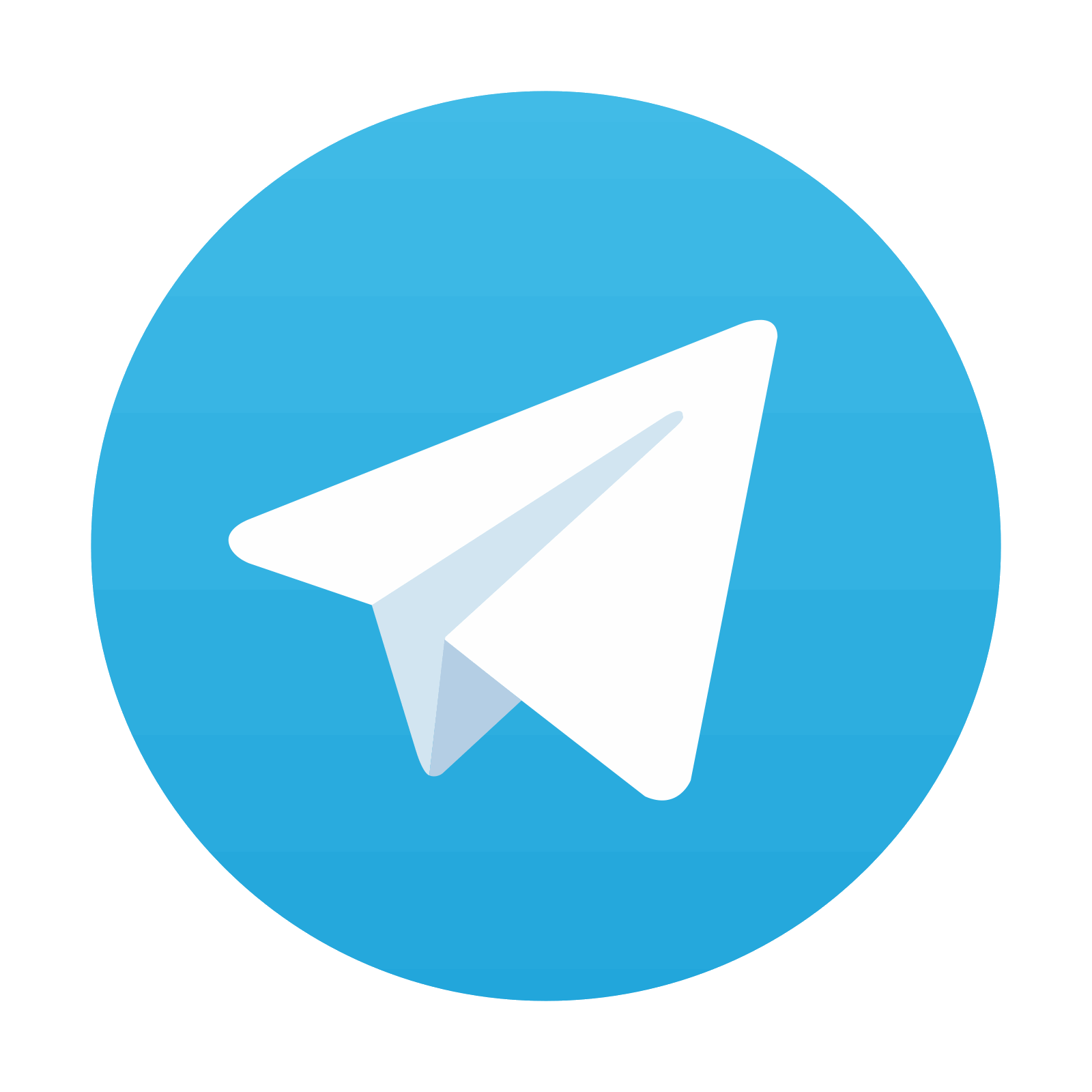
Stay updated, free articles. Join our Telegram channel
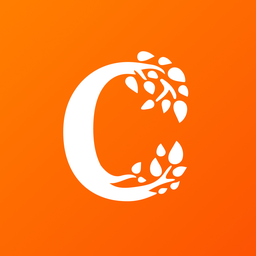
Full access? Get Clinical Tree
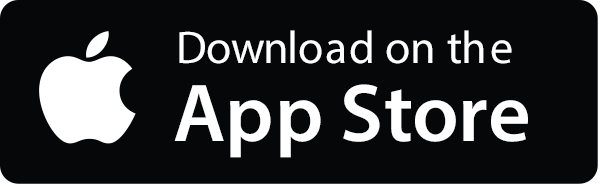
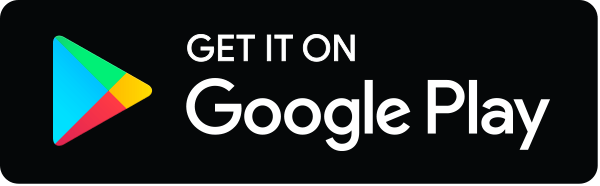