Noninvasive or minimally invasive
Painless
Safe and tolerable
Reversible effect
Modulation of skin permeability
They can be focused on targeted tissues
Possibility of administering macromolecules and nanocarriers (e.g., nanoparticles and liposomes)
Delivery of drugs, but also a mean for sampling biological solutes (e.g., glucose)
More interesting, the combination of chemical and physical/mechanical enhancers has shown a synergistic effect in most of the cases (Mitragotri 2000; Vanbever and Préat 2000; Denet et al. 2004; Motlekar and Youan 2006; Prausnitz and Langer 2008; Banga 2011; Polat et al. 2011b). In this chapter, the enhancement effect of the combined use of sonophoresis, electroporation, and microneedles with chemical enhancers is reviewed.
26.2 Chemical Enhancers and Sonophoresis
26.2.1 What Is Sonophoresis?
Sonophoresis or phonophoresis, defined as the ultrasonically facilitated delivery of drugs to or through the skin, has emerged as one of the most promising physical enhancers (Mitragotri 2013). In this technique, a piezoelectric crystal is responsible for converting electrical energy into acoustic waves. These waves are conducted from the sound source to the skin by a coupling medium (Herwadkar and Banga 2012). Ultrasound has been widely used in sports medicine since the 1950s. As shown in Table 26.2, ultrasound can be classified, according to the frequency range. In the case of the skin, transport enhancement has shown to be more efficient with low-frequency ultrasound (<100 kHz). Low-frequency high-intensity ultrasound waves (2–50 W/cm2) can form pores in the SC, allowing the transdermal delivery of macromolecules up to 48 kDa (Herwadkar and Banga 2012). In general, skin permeability increases with decreasing frequency and with increasing exposure time and intensity (Khafagy et al. 2007). Ultrasound can be applied continuously or in a sequential mode (pulsed mode). Continuous high-frequency ultrasound has shown to be less effective for transdermal drug delivery (Monti et al. 2001). Furthermore, temperature rises faster and more intense in the continuous mode (Machet and Boucaud 2002). Excellent reviews of sonophoresis have been published in the last years (Machet and Boucaud 2002; Lavon and Kost 2004; Mitragotri and Kost 2004; Haar 2007; Ogura et al. 2008; Polat et al. 2010; 2011b).
Ultrasound | Frequency range |
---|---|
Therapeutic/diagnostic | ≥0.7 MHz |
Low frequency | 20–100 kHz |
High frequency | 1.0–3.0 MHz |
Sonophoresis was used for the first time in the 1950s to enhance the absorption of hydrocortisone. In general, low-frequency sonophoresis has shown to enhance the transdermal transport of drugs, reducing the associated lag time, being useful for the local and systemic delivery of drugs. Sonophoresis can be applied in two manners: (i) simultaneously with the drug and (ii) prior to drug delivery, pretreating the skin (Mitragotri and Kost 2004).
Within certain ultrasound intensity range, a direct dependence between intensity and permeability enhancement has been observed, i.e., enhancement increases from a threshold intensity until a maximum intensity value, beyond which, no further increase is observed. Besides ultrasound energy intensity, enhancement also depends on frequency, duty cycle, and application time. Due to the direct relationship between conductance and skin permeability, the effect of sonophoresis on skin permeabilization can be determined by conductance measurements. Ultrasound exerts a dose-dependent effect on skin permeability, with a range of safety: Low-frequency sonophoresis at low intensities (e.g., 2.5 W/cm2) appears to be safe and tolerable, producing minimal urticarial reactions, with no detectable changes in the structure of human skin. Therefore, suitable conditions must be carefully selected, in order to avoid undesirable effects. A low-frequency portable ultrasound device (SonoPrep Ultrasonic Skin Permeation System, Sontra Medical Corporation) for skin permeabilization has been approved by the US Food and Drug Administration (FDA) (Mitragotri 2004; Alexander et al. 2012).
As mentioned, important experimental variables include:
- (i)
Ultrasound duty cycle, i.e., the time that ultrasound is on. In this sense, ultrasound application can be continuous or in pulses (e.g., 10 % corresponds to 0.1 s ON and 0.9 s OFF). Ultrasound pulsing is preferred since it allows heat dissipation, decreasing thermal effects. A greater effect of lignocaine on sensory blockade was found with pulsed ultrasound (Shipton 2012).
- (ii)
Distance between the ultrasound horn and the skin. The horn can be in direct contact with the skin, or as far as 4 cm from the skin surface.
- (iii)
Treatment time. It varies greatly from few seconds to a few minutes or even hours to days.
- (iv)
Composition of the ultrasound coupling medium. The coupling medium is typically an aqueous formulation that can contain a drug (simultaneous treatment protocol) or a chemical enhancer, e.g., a surfactant (pretreatment protocol) (Polat et al. 2012).
Low-frequency sonophoresis has shown to effectively enhance the transport of high molecular weight drugs, such as heparins, insulin, γ-interferon, and erythropoietin (Mitragotri et al. 1995; Mitragotri et al. 1996; Mitragotri and Kost 2004; Motlekar and Youan 2006; Herwadkar and Banga 2012). Apparently, cavitation (formation and immediate implosion of bubbles in a liquid) opens large pores and channels in the lipid layers of the SC, providing a less tortuous path for the transport of macromolecules. However, it is important to point out that in the case of the simultaneous application of ultrasound and the drug, the effect of ultrasound on protein conformation and/or activity should be determined (Khafagy et al. 2007).
Other applications of low-frequency ultrasound include (Mitragotri et al. 2000; Mitragotri and Kost 2004; Hosseinkhani and Tabata 2005; Frenkel 2008; Mitragotri 2013):
Extraction of different analytes from the skin’s interstitial fluid for diagnostic purposes, e.g., glucose, albumin, calcium, urea, triglycerides, lactate, and dextran.
Enhancement of drug penetration into the brain by disruption of the blood-brain barrier.
Enhancement of the transcorneal permeation of drugs.
Acceleration of clot dissolution (sonothrombolysis).
Delivery of drugs and genes to solid tumors.
26.2.2 Mechanisms of Action of Sonophoresis
Cavitation. Mechanism of low-frequency sonophoresis is mainly related to the formation and collapse of gaseous cavities (cavitation). Collapse of cavitation bubbles can cause structural alteration of tissues due to the shock wave generated. The radius of the cavitation bubbles has a relationship with the frequency and pressure amplitude. Low-frequency ultrasound produces a greater cavitation effect with respect to therapeutic ultrasound (high intensity), resulting in a higher enhancement effect (Mitragotri et al. 1996; Monti et al. 2001). The shock waves generated by bubbles disrupt the intercellular lipids of the SC, causing conformational changes, increasing the porosity of the skin, and thus promoting the diffusion of a solute (Ueda et al. 1996; Joshi and Raje 2002; Mitragotri and Kost 2004). It has been suggested that cavitation is the primary mechanism of enhancement in highly perturbed skin regions, known as localized transport regions (LTRs) (Polat et al. 2011a).
Although cavitation is the main enhancement mechanism, non-cavitational mechanisms have also been reported:
Convection. Simultaneous application of a drug formulation and ultrasound enhances drug transport in two ways: (i) by structural changes in the lipids of the skin and (ii) through convection-related mechanisms. Contradictory results are reported: Some authors have found no effect of convection on skin permeability, while other studies have suggested an important role of convection when using low-frequency sonophoresis (Polat et al. 2011b).
Thermal effects. Temperature increases are directly proportional to ultrasound intensity and duty cycle. Although it is expected that high temperature increases result in enhanced transdermal transport, the harmful side effects observed with significant increases in temperature have led to a strict temperature control that minimizes heating. Rise in temperature is one of the major factors that increase percutaneous absorption in the 1–3 MHz frequency range and in continuous mode (Machet and Boucaud 2002).
Lipid extraction. According to Alvarez-Roman et al. (2003), low-frequency sonophoresis is able to extract an important amount of the SC lipids (about 30 %).
Stratum corneum hydration. Ueda et al. (1996) reported that ultrasound increases the fraction of aqueous region in the SC, increasing the diffusivity of drugs.
Apparently, transfollicular pathways are more susceptible to ultrasonic enhancement than are transcellular processes (Meidan et al. 1995). An important fact is that sonicated skin recovers its original barrier properties, with only slight histological changes. Some authors (Monti et al. 2001; Machet and Boucaud 2002) reported an immediate recovering of skin barrier once turning off sonication, while Lavon and Kost (2004) argued that a single short application (less than 2 min) of ultrasound may enhance skin permeability over several hours, recovering its normal value by 24 h. A greater enhancement activity of ultrasound has been found for hydrophilic molecules with respect to less hydrophilic ones (Monti et al. 2001).
26.2.3 Synergistic Enhancement Effect of Sonophoresis and Chemical Enhancers
In this section, studies related to the simultaneous use of sonophoresis and chemical enhancers are reviewed. Table 26.3 represents some of these results. However, it is important to mention that sonophoresis has also been combined with other physical or mechanical enhancement methods, such as microneedles (Chen et al. 2010; Han and Das 2013; Han and Das 2015; Pamornpathomkul et al. 2015) and iontophoresis (Ueda et al. 1996; Le et al. 2000; Fang et al. 2002; Watanabe et al. 2009). This kind of treatment has shown to greatly enhance the delivery rate of large molecular weight compounds into the skin. Furthermore, combination of US with some carriers has shown a greater permeation than when applied separately, e.g., polymeric, lipid-based nanoparticles, micelles and elastic liposomes (Husseini and Pitt 2008; Kasetvatin et al. 2015).
Table 26.3
Examples of the synergistic effect of chemical enhancers and ultrasound on skin permeability
Chemical enhancer | Drug | Ultrasound conditions | Animal model | Skin permeability | Reference |
---|---|---|---|---|---|
Linoleic acid/ethanol (1:1), polyethylene glycol 200 dilaurate, isopropyl myristate, glycerol trioleate, ethanol/pH 7.4 phosphate buffer (1:1) | Corticosterone | 1 MHz, 1.4 W/cm2 | Human cadaver skin | Ethanol/linoleic acid: 87-fold increase Ethanol/linoleic acid + US: 14.4-fold increase | Johnson et al. (1996) |
Pretreatment of the skin with US, Tween® 20 (1 %) as donor solution, constant current iontophoresis (0.1 mA/cm2) | Benzoate anion (BA) | 150 kHz, 111 W/cm2, irradiation area: 3.14 cm2 | Excised abdominal hairless rat skin | Increase of BA flux (~1.5 folds) with 60 min US pretreatment. Leaching of sterols and ceramides. Disordering of lipid packing. US enhances stratum corneum hydration | Ueda et al. (1996) |
Laurocapram (Azone®) or oleic acid (1 % v/v) | Hydrocortisone | 1.1 and 3.3 MHz | In vitro rat skin | Azone® and US (3.3 MHz, 1.5 W < 7 cm2) EF ~ 3. No synergistic effect was observed with oleic acid | Meidan et al. (1998) |
Benzalkonium chloride, oleyl alcohol, and α-terpineol (1 % v/v of each enhancer), all of them separately in propylene glycol | Morphine (MOR) and caffeine (CAF) | 40 kHz (<0.5 W/cm2) 1.5–3.0 MHz (only for caffeine) | Male hairless mice in vitro | US and chemical enhancers were used separately No effects of US on lag times No effect of high-frequency US on CAF permeation Greater effect of US on MOR (EF = 39.8 with 40 kHz, 440 W/cm2) than on CAF (EF = 3.81 with 40 kHz, 438 mW/cm2) The same enhancing effect on MOR for oleyl alcohol and US (EF = 32–39) | Monti et al. (2001) |
5 % d-Limonene/ethanol | Ketorolac tromethamine | 1 MHz, 1–3 W/cm2 | In vitro rat skin | Pretreatment with limonene and US at 3 W/cm2 significantly enhances drug permeation when compared with passive flux | Tiwari et al. (2004) |
SLS (1 %) | Calcein | 20 kHz | In vitro pig skin | Synergistic enhancement (EF = 35) when applied simultaneously | Lavon et al. (2005) |
Menthol (10 %), SLS (0.05 %), Azone® (5 %), N-methylpyrrolidine (5 %), DMSO (5 %), and electroporation [1 pulse/20 s (3 ppm) during 20 min (110 V, pulse length 300 ms)] | Cyclosporine A (CsA) | 20 kHz | In vitro rat skin | Combination of US with Azone® and SLS yields the highest amount in the receptor medium (2.82 and 3.74 times than the passive diffusion, respectively) US combined with electroporation was not effective. Azone® + US + electroporation increased the amount of CsA retained in the skin, but were lower than US + Azone® | Liu et al. (2006) |
1 % SDS, pretreatment with a liposomal formulation | Model antigen (bovine serum albumin) | 20 kHz, 30 % amplitude, duty cycle 50 % (0.5 s on, 0.5 s off), sonication time 2 min | In vitro: Full-thickness rat skin In vivo: Male Wistar rats | In vitro: Reduction in antigen permeation due to liposome adsorption and fusion with skin surface, when PBS was used as coupling medium. Important skin damage with SDS In vivo: Reduced TEWL (with PBS), increased TEWL (with SDS) | Dahlan et al. (2009) |
SLS (1 %) | Sucrose, Au nanoparticles, quantum dots | 20 kHz, 7.5 W/cm2, pulse (5 s on, 5 s off) | In vitro Human and pig skin (full and split thickness) | Split-thickness pig skin is a good option to perform US/SLS experiments in the case of hydrophilic macromolecules | Seto et al. (2010) |
SLS (1 %) | Cationic, neutral, and anionic quantum dots (QDs) | 20 kHz, 7.5 W/cm2, pulse (5 s on, 5 s off) | Pig split-thickness skin | Permeation through US/SLS-treated skin, reaching the receiver compartment (mainly for cationic particles). QDs did not reach the receiver chamber for untreated skin | Lopez et al. (2011) |
SLS (1 %) | Calcein | 20, 40, and 60 kHz; 7.5 W/cm2, duty cycle 50 % (5 s on, 5 s off) | Skin harvested from the back and flank of female Yorkshire pigs | Calcein permeability decreases with increasing US frequency. In the LTRs regions, pore radii in the skin increase with decreasing frequency. In the non-LTRs, pore radii are independent of US frequency | Polat et al. (2011a) |
SLS (1 %) | Sucrose | US/SLS and US. 20 kHz, 7.5 W/cm2, duty cycle 50 % (5 s on, 5 s off). Samples were treated until they reached currents between 5 and 200 μA | Skin harvested from the back and flank of female Yorkshire pigs (full-thickness skin, FTS, and split-thickness skin, STS) | US-treated skin required 5–12-fold longer treatment times than the US/SLS-treated skin to reach similar skin electrical resistivity | Polat et al. (2011c) |
Chemical enhancers are known to permeabilize the skin by different mechanisms such as lipid fluidization, lipid extraction, or protein denaturation. It has been demonstrated that a combination of penetration enhancers showing different modes of action frequently exerts a synergistic effect on drug permeation (Mitragotri 2000). This is important, since it can enable a reduction in the dose/time of exposition to the enhancer in order to achieve the desired effect, reducing or even avoiding undesirable effects related to high concentrations of chemical enhancers. The synergistic effect of ultrasound and chemical enhancers is attributed to cavitation that may induce and promote the dispersion of chemical enhancers with SC lipids (Lee et al. 2010).
Johnson et al. (1996) showed that corticosterone flux was enhanced by a factor of 903 (skin permeability increase, 8.7) when the skin was treated with ethanol/linoleic acid (1:1) in combination with ultrasound. The same formulation enhanced the flux through the skin by a factor of 13,000 (skin permeability increase: 14.4), when combined with therapeutic ultrasound (1 MHz, 1.4 W/cm2). The authors attributed this effect to a lipid fluidization or to the formation of a separate bulk of lipid domain in the SC. A synergism was also observed for the simultaneous application of ultrasound and laurocapram (Azone®). The authors suggested an enhancement in laurocapram diffusion into the skin due to ultrasonic thermal effects (Meidan et al. 1998). The combined application of oleic acid and low frequency ultrasound was shown to enhance the penetration of lanthanum nitrate (LaNO3) into the viable epidermis through an intracellular pathway. LaNO3 was found uniformly distributed both in the intracellular and intercellular domains (Lee et al. 2010). These authors also found a synergistic effect in LaNO3 when ultrasound was applied on tape-stripped skin.
A significant synergistic effect has been reported for the combined use of low-frequency ultrasound and surfactants. Simultaneous application of ultrasound and sodium lauryl sulfate (SLS) significantly increases the permeability and conductivity of the skin (Lavon et al. 2005). In fact, addition of SLS to the coupling medium has shown a linear increase in skin permeability in an SLS concentration range of 0–1 % (Mitragotri et al. 2000). The concentration of 1 % SLS in a solution is approved by the FDA for many cosmetic and pharmaceutical products (Lavon et al. 2005). SonoPrep® (Sontra Medical Corporation) is an ultrasonic skin permeation system that enhances the effectiveness of topical anesthetics, shortening the onset of topical anesthetics action (Kim et al. 2012). This system operates with 1 % SLS solution, showing no skin irritation (Boucaud 2004; Lavon et al. 2005).
Mitragotri et al. (2000) found a dramatic increase in mannitol permeability (200-fold enhancement) when 1 % SLS and ultrasound are combined. The authors also reported a reduction of the threshold ultrasound energy to produce a change in skin impedance, from 141 J/cm2 (without SLS) to 18 J/cm2 (with 1 % SLS). A reduction of treatment times, higher connectivity of lacunar regions of the SC, as well as a lower perturbation of the skin have been also demonstrated when SLS is used with sonophoresis, compared to sonophoresis alone (Polat et al. 2012). The synergistic enhancement of ultrasound and SLS was clearly demonstrated by Lavon et al. (2005), who found an enhancement ratio of ~ 35, while when applied separately, enhancement ratios were 4 for SLS and 12 for ultrasound. Polat et al. (2011a) studied the permeability of calcein at three frequencies (20, 40, and 60 kHz), with important contribution to the following points:
- (a)
Calcein showed a much greater permeability through the localized transport regions (LTRs) than through all the other skin regions (i.e., non-LTRs and untreated skin). Non-LTRs were also more permeable than the native skin (untreated). In this case, heating or microstreaming could increase the flux of SLS into the skin (almost twofold SLS penetrated into non-LTRs than into native skin).
- (b)
Calcein permeability was dependent on ultrasound frequency, since permeability decreased with increasing ultrasound frequency.
- (c)
Pore radii in the LTRs exhibited a decrease with increasing ultrasound frequency (>300, 276, and 161 Å for 20, 40, and 60 kHz, respectively). Pore radii were independent of ultrasound frequency in the non-LTRs (~18 Å vs. ~ 13 Å in native skin).
- (d)
Permeability of calcein in LTRs was of similar magnitude to the permeability in the dermis, suggesting that the barrier properties of the epidermis were defeated with the sonophoretic treatment.
In order to investigate the synergism between ultrasound and SLS, Polat et al. (2011c) utilized two skin models, pig full-thickness skin (FTS) and pig split-thickness skin (STS), with two kinds of treatments, ultrasound (US) alone and ultrasound with SLS (US/SLS). Samples were treated with ultrasound until they reached currents between 5 and 200 μA. The authors studied the treatment time required to attain specific levels of skin electrical resistivity and found that US/SLS treatment reduced dramatically the time necessary to reach similar skin electrical resistivity values, with respect to the US-treated samples. Furthermore, the presence of SLS induced a more reproducible skin perturbation.
Sodium dodecyl sulfate (SDS) at 1 % (w/v) has also been used as the coupling medium, to investigate the influence of liposomes on in vitro antigen permeation and on in vivo skin barrier properties, using rats as animal models. The authors found important skin damage attributed to the synergistic effect of ultrasound and SDS (Dahlan et al. 2009). Similar findings were previously reported by Mitragotri et al. (2000) and Tezel et al. (2002).
Physicochemical characteristics of surfactants determine their action. Apparently, ionic surfactants are better enhancers than nonionic surfactants, and 14-carbon tail length in the surfactant molecule is the optimum to attain a synergistic effect with low-frequency ultrasound (Tezel et al. 2002).
Surfactant–sonophoresis synergism can be attributed to:
- (i)
The effect of surfactant on ultrasound-related phenomena. Being adsorbed at interfaces, surfactants reduce the surface tension of aqueous solutions. Surface tension has an important effect on the oscillation of a cavitation bubble, causing an increase in the rate of expansion and a decrease in the rate of compression as the surface tension decreases. Furthermore, charged surfactants stabilize cavitation bubbles by electrostatic repulsion, resulting in a great number of small cavitation bubbles.
- (ii)
The effect of ultrasound on surfactant penetration and interaction with SC lipids (Tezel et al. 2002; Polat et al. 2011b). Sonophoresis favors the deep penetration of surfactants into the skin, allowing them to induce a greater fluidization of SC lipids (Polat et al. 2012). Ueda et al. (1996) found that ultrasound significantly increased extraction of sterols and ceramides when 0.1 % Tween® 20 was used as donor solution, lowering skin impedance and therefore increasing SC diffusivity. When distilled water was used as donor solution, leaching of lipids was not detectable. SLS has also the ability to solubilize or extract lipids (Polat et al. 2011a).
- (iii)
Surfactants can denature keratin fibers of corneocytes (Polat et al. 2011a).
- (iv)
Surfactants can expand lacunar regions, thereby creating less tortuous and more direct permeation pathways (Polat et al. 2011c).
- (v)
The simultaneous use of ultrasound and surfactants such as SLS is able to provoke changes in skin pH profile of the SC. Sonication can result in a pH reduction of the SC. In this acidic environment, SLS is in the form of free acid, being more soluble in the SC lipids and, therefore, more available to act as enhancer (Lavon et al. 2005).
The physical phenomena involved in the combined use of sonophoresis and permeation enhancers have been well explained (Lee et al. 2010; Polat et al. 2012; Azagury et al. 2015). The mechanisms are related to the following aspects:
- (i)
The ability of ultrasound to increase penetration and dispersion of permeation enhancers. This effect is explained by a convective flux induced by the collapse of acoustic cavitation microjets, which allows direct deposition of enhancers into the skin.
- (ii)
A greater synergism has been observed between amphiphilic enhancers (like SLS) and sonophoresis than with non-amphiphilic enhancers (e.g., propylene glycol), due to the adsorption of amphiphilic monomers to the surface of the cavitation bubbles.
- (iii)
The enhancement is even greater when binary mixtures of surfactants are used. This can be attributed to a more efficient adsorption or packing of two surfactants on the surface of cavitation bubbles.
- (iv)
Only surfactant monomers can be adsorbed onto the surface of cavitation bubbles. Synergism depends on monomer concentration. Therefore, in the case of surfactants, below their critical micellar concentration (CMC), a further enhancement of their flux is expected due to adsorption of monomers onto the surface of cavitation bubbles. However, above CMC, adsorbed monomer concentration is constant due to the inability of micelles to be adsorbed. Therefore, in the case of surfactant mixtures, a decrease of the CMC may lead to antagonism, while an increase in CMC may explain a stronger synergism with sonophoresis (Polat et al. 2012).
- (v)
Combinations of mixtures of chemical enhancers acting as “extractors” (i.e., able to extract membrane lipids) and “fluidizers” (i.e., able to disrupt lipid domains) with ultrasound, resulted in synergy. Combinations of chemical enhancers from the same group may even produce an antagonistic effect.
It is interesting to mention that in some cases, a greater enhancement activity has been reported for chemical enhancers alone than for low-frequency ultrasound alone. In this sense, Monti et al. (2001) found a higher enhancement effect on caffeine permeation with oleic acid/propylene glycol than with low-frequency ultrasound.
26.3 Chemical Enhancers and Electroporation
26.3.1 What Is Electroporation?
Electroporation was originally used to transfect cells with macromolecules such as DNA by altering their cell membranes, afterward it has been applied in tissues (e.g., for gene therapy) to reversibly permeabilize them (Denet et al. 2004). The investigation of electroporation for drug delivery began in the 1990s (Banga 2011). In recent years, electroporation has been proposed for delivery of DNA vaccines and for electrochemotherapy, which consists of applying high-voltage pulses to permeabilize tumor cells against cytotoxic drugs (Denet et al. 2004).
Electroporation or electropermeabilization is an electric enhancement technique that involves application of high-voltage pulses (50 to 1500 V) for very short durations of time (micro- to milliseconds) to induce the transitory structural perturbation of lipid bilayer membranes (Higaki et al. 2003; Brown et al. 2006; Banga 2011; Alexander 2012; Wong 2014). These intense electric charges generate structural rearrangements of the cell membrane and electrical conductance and changes of membrane conductance leading to a temporary loss of semipermeability of cell membranes (Banga 2011). The electroporation of the lipid bilayers of the SC causes the formation of transient aqueous pathways or electropores through it. These electropores allow drugs to penetrate more readily (Denet et al. 2004; Wong 2014; Ita 2015).
26.3.2 Factors Influencing Drug Delivery by Electroporation
The electroporation mechanism has been comprehensively reviewed by several authors (Weaver and Chizmadzhev 1996; Weaver et al. 1999; Pliquett 1999; Sen et al. 2002a; Denet et al. 2004; Becker et al. 2014). The most important factors in the enhancement of percutaneous absorption of drugs by electroporation are the selection of the electrical parameters: pulse waveform (exponential decay or square wave), pulse voltage, pulse length, number of pulses, and spacing between pulses ). (Denet et al. 2004; Banga 2011; Zorec et al. 2015). Usually, the skin transport of the solutes improves when the applied voltages are increased (Higaki et al. 2003). The application of high-voltage electric pulses may be accompanied by temperature changes in the pulsing medium. A long-duration pulse could increase skin irritation (Banga 2011). The transdermal voltage is only a fraction of the voltage applied across the electrodes (ca. 10–50 %) since a significant voltage drop occurs within the electroporation system (Denet et al. 2004).
Furthermore, physicochemical properties of the drug and drug concentration can affect the transdermal drug delivery by electroporation (Vanbever and Préat 2000). The pKa of the drug and the pH of the delivery solution influence the electric charges of the molecule to be delivered. Neutral molecules can diffuse through the permeabilized skin (passive diffusion); however, their transport is lower than the transport of charged molecules during pulses (Denet et al. 2004). Electroporation has been successfully used to enhance the skin permeability of molecules with different lipophilicity and size, including biopharmaceuticals with a molecular weight greater than 7 kDa, the current limit for iontophoresis (Mathur et al. 2010). Small- and moderate-sized molecules such as fentanyl, timolol, metoprolol, mannitol, calcein and cyclosporine A, etc., have been transported transdermally by electroporation (Denet et al. 2004). The influence of the molecular weight of the permeant on transdermal and topical transport by skin electroporation was first evaluated by Lombry and coworkers (Lombry et al. 2000). Fluorescein isothiocyanate (FITC) and fluorescein isothiocyanate–dextran (FD) macromolecules with increasing molecular weight (4.4, 12, and 38 kDa) were used as fluorescent permeant models. The transport of FITC and FD across hairless rat skin in vitro and their distribution in the skin was explored by confocal scanning laser microscopy. The results showed that it was feasible to deliver large macromolecules (at least up to 40 kDa) across the skin by electroporation. Concurrently, authors demonstrated that the macromolecule dextran enhanced FITC transport.
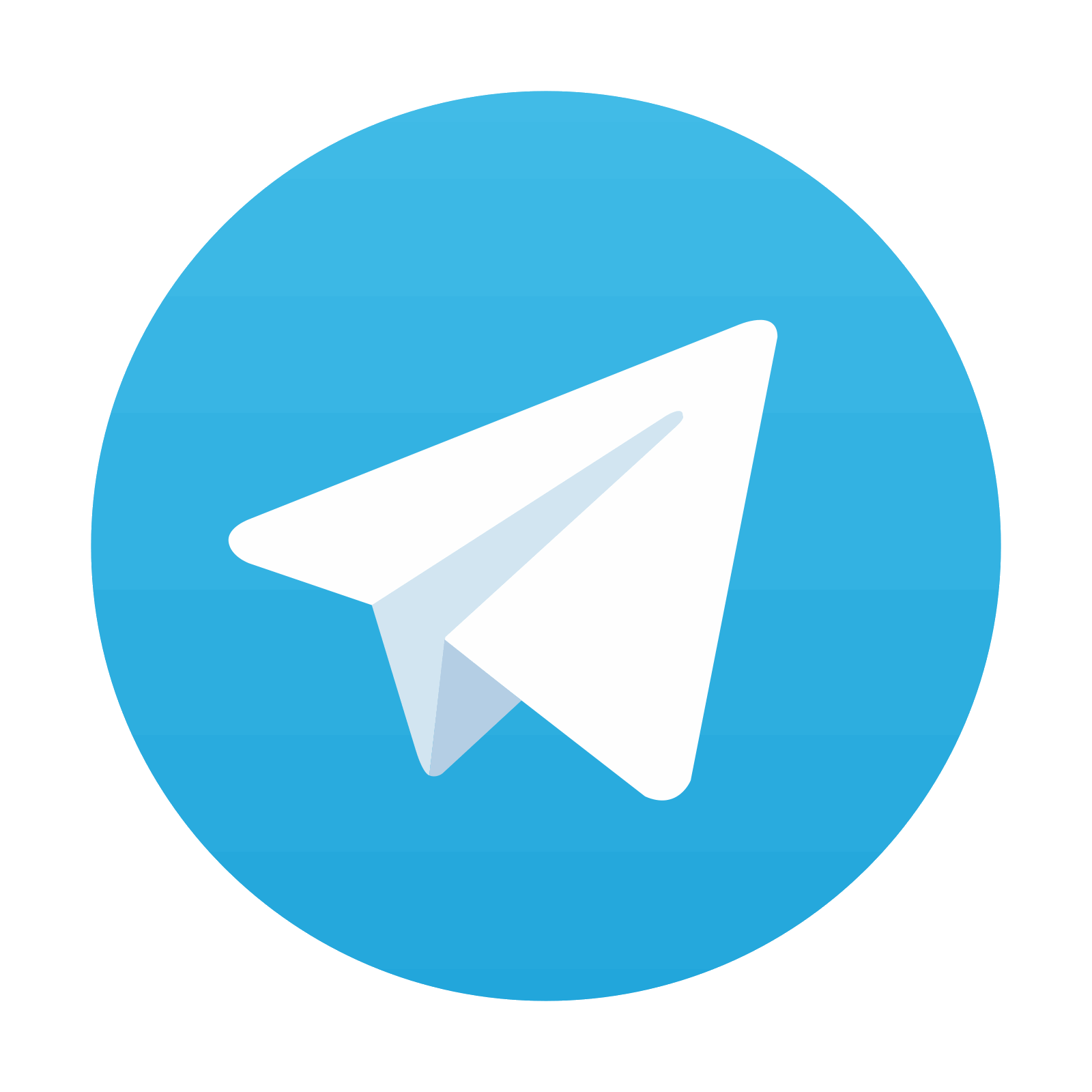
Stay updated, free articles. Join our Telegram channel
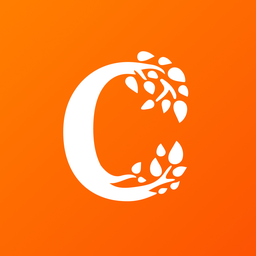
Full access? Get Clinical Tree
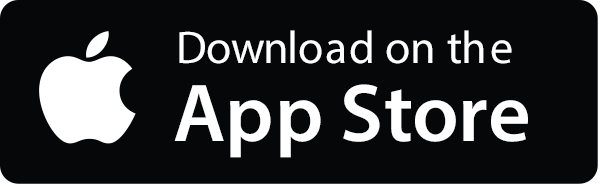
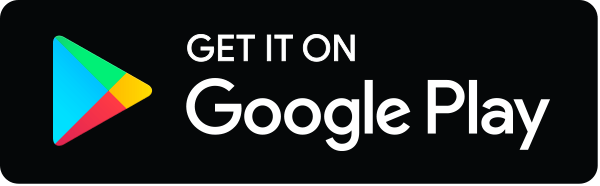