© Springer-Verlag Berlin Heidelberg 2017
Nina Dragicevic and Howard I. Maibach (eds.)Percutaneous Penetration Enhancers Physical Methods in Penetration Enhancementhttps://doi.org/10.1007/978-3-662-53273-7_2828. Cutaneous DNA Immunization: Enhancing Penetration by Hair Follicle Modification or Microneedle Application
(1)
Dell Pediatric Research Institute, The University of Texas at Austin, Austin, TX, USA
Keywords
Hair folliclesMicroneedlesPlasmidCutaneous immunizationNanoparticles28.1 Introduction
Almost all vaccines, with a few exceptions, are administered by intramuscular (IM) or subcutaneous (SC) injection using hypodermic needles. While needle-based vaccination has led to tremendous advancement in controlling many infectious diseases, this technique comes with several risks such as potential infection at the site of injection, spread of infectious needles and syringes as well as reuse of non-sterile needles and syringes, and discomfort to the patient. Thus, alternate means of vaccine delivery have been explored to attain a more effective, safer, and patient-friendly vaccination method (Levine and Sztein 2004).
For a long time, skin was considered impermeable for large molecules such as protein antigens, but studies performed by Glenn et al. demonstrated that topical application of protein antigens, such as diphtheria or tetanus toxoids, admixed with cholera toxin (CT) onto a skin area stimulated specific immune responses to the protein antigens in a mouse model (Glenn et al. 1998). A similar result was obtained when the heat-labile enterotoxin (LT) of Escherichia coli (Beignon et al. 2001) or its mutants LTK63 and LTR72 were used as adjuvants (Beignon et al. 2002). The preliminary results of a clinical trial conducted with human volunteers also demonstrated that topical application of an LT patch onto the skin induced anti-LT immunoglobulin G (IgG) and immunoglobulin A (IgA) responses (Glenn et al. 2000). After these ground-breaking studies, cutaneous immunization (i.e., immunization by applying vaccines or antigens topically onto the skin) continuously evolved as a noninvasive technique that benefits from the skin immune system.
The skin is the largest organ of the human body. It acts as a physical barrier between body and the outer environment. The skin is mainly composed of two layers, the epidermis and dermis. The epidermis is made up of stratum corneum and viable epidermis. The stratum corneum is the outer most layer, which is 30–50 μm thick and composed of dead cells called corneocytes embedded in a highly organized lamellar structure formed by intercellular lipids. The barrier property of the skin resides in the stratum corneum layer, which plays a crucial role in shielding the body from the external environment. Just below the stratum corneum is the viable epidermis, which is 200–250 μm thick. The main cell type of the epidermis are keratinocytes; however, melanocytes, Merkel cells, and Langerhans cells (LCs) are also present, although less abundantly. The dermis is 2–3 mm thick. Cells present in the dermis are fibroblasts, mast cells, and dendritic cells (DCs). The dermis layer also has blood vessels, lymph vessels, nerves, and an abundant level of collagen fibers. Below the dermis, there is a layer of the subcutaneous fat tissue (Karande and Mitragotri 2010; Bos and Meinardi 2000; Schaefer and Redelmeier 1996).
Other than the physical barrier function, skin is also a part of the immune system. It is highly accessible and has a unique immunological characteristic. Keratinocytes and LCs of the epidermis layer, fibroblasts, DCs, and mast cells of the dermis layer, and T and B lymphocytes of the skin-draining lymph nodes together form the skin’s whole immunization network (Karande and Mitragotri 2010). Skin has a rich population of DCs, which provide effective immunity against antigens coming through the skin. LCs are the primary DCs in the epidermis that migrate from bone marrow to skin and play a vital role in immune surveillance and antigen presentation. DCs act as efficient and potent antigen-presenting cells (APCs) for the induction of adaptive immunity (Glenn and Kenney 2006). These APCs (i.e., LCs and DCs) capture and process antigens at the site of administration and migrate to local draining lymph nodes, where they mature and mediate the activation of B and T cells (Banchereau and Steinman 1998). Conventional vaccination by hypodermic needle-based injection bypasses the APC-rich skin immune system and delivers antigens into the less APC-populated muscle or subcutaneous tissues. Delivery of antigens into skin layers, where a large number of epidermal LCs and dermal DCs are present, can potentially induce a greater immune response (Glenn and Kenney 2006; La Montagne and Fauci 2004). In fact, data from many clinical studies have shown that intradermal administration of influenza vaccine requires a very low dose, relative to intramuscular injection, to produce a similar or even stronger immune response (Kenney et al. 2004; Belshe et al. 2004). Another major advantage of cutaneous immunization is its potential safety. Cutaneous immunization minimizes the distribution of antigens and adjuvants inside the body, which may produce slight toxicity if comes directly in contact with the systemic circulation (Ponvert and Scheinmann 2003).
Recombinant DNA technology has revolutionized vaccine development. DNA vaccine, based on recombinant DNA technology, comprises a number of features that make it an attractive alternative over the conventional live attenuated, inactivated, or protein-based vaccines. DNA vaccine manufacturing is simple and rapid, and plasmid DNA is very stable during storage. DNA vaccine has the potential to generate strong cellular and humoral immune responses and is well tolerated by patients (Patil et al. 2005). Plasmid DNA can be easily manipulated to incorporate multiple antigen genes in the same plasmid (Phillips 2001). Plasmid DNA vaccine can also overcome certain safety concerns associated with live vaccines, such as reversion risks and potential spread to unintended individuals (Shedlock and Weiner 2000; Ruprecht 1999). Additionally, plasmid DNA itself has some adjuvant activity because of the presence of unmethylated CpG motifs (Cornelie et al. 2004; Ban et al. 2000).
DNA vaccine came in existence in the 1990s. In a very first study, Tang et al. demonstrated that immune response can be stimulated by administering a protein-encoding gene into the skin of mice. The authors reported the delivery of plasmid DNA containing human growth hormone gene into the skin using a gene gun in an attempt to express human growth hormone for gene therapy. Through this study, the authors pointed out that plasmid DNA may be used to produce antibodies and may offer a unique method for vaccination (Tang et al. 1992). One year later, Ulmer et al. reported that intramuscular injection of naked plasmid DNA can induce immune responses against influenza viral antigens in mice (Ulmer et al. 1993). In the same year, Fynan et al. demonstrated that other than IM injection, epidermal, mucosal, and intravenous routes can also be used for the administration of DNA vaccine. It was concluded that vaccination with plasmid DNA expressing influenza virus hemagglutinin glycoprotein by gene gun delivery into the epidermis or by administration of plasmid DNA to nasal mucosa is most promising (Fynan et al. 1993). After these breakthroughs, numerous studies had been performed using various plasmid DNA formulations and delivery methods, and altogether, they provided solid evidence that DNA vaccine is potentially viable. In 1999, Shi et al. reported that noninvasive immunization through mouse skin with plasmid DNA induced an immune response against the protein encoded by the plasmid (Shi et al. 1999). Watabe et al. later confirmed that the stratum corneum is the major barrier for a successful cutaneous DNA immunization. The authors reported that applying plasmid DNA that encodes an influenza viral antigen onto intact mouse skin could not induce a high level of immune response, even when a very large amount of plasmid DNA was used. However, immunization with the same plasmid after the removal of the stratum corneum by tape stripping with fast active adhesive glue significantly improved the resultant immune responses, to a level similar to that after IM injection (Watabe et al. 2001), clearly demonstrating the need to overcome the stratum corneum barrier for cutaneous DNA immunization to induce strong and effective immune responses.
28.2 Techniques to Overcome the Stratum Corneum Barrier
The stratum corneum is the principle barrier for skin penetration. This layer has made it very challenging to deliver high molecular weight hydrophilic molecules such as proteins, peptides, and plasmid DNA into or across the skin (Bos and Meinardi 2000). The stratum corneum is predominantly composed of dead corneocytes surrounded by lipid layers (Elias 1983); it offers an extensive barrier to small as well as large hydrophilic compounds. Only certain molecules with a molecular weight under 500 Da can be delivered passively through the stratum corneum (Prausnitz et al. 2004). To overcome the stratum corneum barrier and to deliver hydrophilic high molecular weight molecules such as plasmid DNA into the skin, various alternative approaches have been explored, including chemical and physical techniques (Prausnitz et al. 2009).
28.2.1 Physical Techniques
28.2.1.1 Iontophoresis
Iontophoresis is the transdermal delivery of therapeutic molecules in ionic form. It involves the application of electrical current, typically a few milliamperes, across the skin to deliver hydrophilic and charged molecules. In this technique, an electrical current is applied through two electrodes across the skin surface. This allows the delivery of drug in a controlled fashion because the amount of drug delivered across the skin is directly proportional to the amount of charge passed (Ahad et al. 2010). Examples of US Food and Drug Administration (USFDA)-approved iontophoretic systems include those that deliver lidocaine to induce local anesthesia (Kim et al. 1999), tap water for the treatment of hyperhidrosis (Holzle and Alberti 1987), and pilocarpine to induce sweating (Berner and Dinh 1998). Plasmid DNA has also been delivered into the skin for immunization using this method; however, this method is not very commonly used (Vandermeulen et al. 2007).
28.2.1.2 Electroporation
Electroporation is another technique that includes the use of transmembrane voltage produced by electric pulses to create reversible pores on the membrane surface. Electroporation temporarily disrupts the lipid bilayer membrane by applying short, high-voltage electrical pulses. It has been shown that electroporation can alter the lipid domain of the stratum corneum (Prausnitz 1996; Subedi et al. 2010), which can help the permeation of small molecules such as fentanyl as well as moderate size molecules such as calcitonin (Denet et al. 2004). Electroporation has also been reported to enhance the permeation of lipophilic (e.g., timolol), hydrophilic (e.g., metoprolol), charged (e.g., heparin), and neutral (e.g., mannitol) molecules (Denet and Preat 2003; Prausnitz et al. 1995; Vanbever et al. 1998). The extent of permeation is dependent upon the magnitude of the applied voltage (Prausnitz et al. 1993). Often, electroporation has been combined with intradermal injection of plasmid DNA for vaccination purpose (Drabick et al. 2001; Heller et al. 2001). For more information about electroporation used for intradermal vaccination, please refer to Chap. 30 in this volume (written by Heller).
28.2.1.3 Sonophoresis/Ultrasound
Ultrasound has been shown to increase drug permeability across skin (Mitragotri and Kost 2000). A less than 100 kHz frequency has been found to enhance the transdermal permeability more efficiently than higher-frequency ultrasounds (Boucaud et al. 2002). Ultrasound of less than 100 kHz frequency causes cavitation, which results in the disruption of the lipid bilayers of the stratum corneum and thus enhances the influx of drugs (Mitragotri 2005; Tanner and Marks 2008). Ultrasound can be applied to the skin either before the application of the drug or simultaneously with the application of the drug (Ogura et al. 2008). This method has been shown to increase skin permeability to various low and high molecular weight molecules such as insulin, heparin, and plasmid DNA (Schratzberger et al. 2002; Endoh et al. 2002; Rao and Nanda 2009). In a study performed by Endoh et al., it was demonstrated that intra-amniotic injection of naked DNA with microbubble-enhanced ultrasound generated a very high level of expression of the DNA in fetal mouse skin (Endoh et al. 2002).
28.2.1.4 Thermal Poration
Heat increases skin permeability by creating pores in the stratum corneum. Heat also affects the microcirculation and blood vessel permeability, which are important factors for the administration of drugs in the systemic circulation (Ahad et al. 2010). Heat technique has been used to deliver conventional drugs and DNA vaccines (Sintov et al. 2003; Bramson et al. 2003). Heat also enhances the skin permeability by increasing drug solubility in patch formulation and within the skin (Hull 2002). FDA-approved Synera® (Nuvo Research Inc., Canada) topical patch (lidocaine and tetracaine) for pain relief is based on this mechanism. The unique heating pod present inside Synera® becomes active once it is removed from the storage pouch. It warms up the area where it is applied, helping enhance the penetration of the anesthetics inside the skin (available at www.synera.com; accessed 11 April 2013).
28.2.1.5 Skin Abrasion
Skin abrasion involves direct removal or disruption of the stratum corneum layer to facilitate the transportation of topically applied compounds (Kumar and Philip 2007). In vitro data have shown that this technique can increase the penetration of angiotensin across the skin by 100-fold compared to untreated human skin (Roberts 1997; Murthy et al. 2003). A study by Lisziewicz et al. showed that skin abrasion combined with DNA vaccine, which was formulated as mannosylated particles, induced a strong immune response to HIV virus in monkeys (Lisziewicz et al. 2005).
28.2.1.6 Needle-Free Jet Injection
Needleless jet injection is a combination of transdermal and parenteral drug delivery methods. The injection devices can be divided into two categories: liquid jet injectors and solid jet injectors. Both injectors deliver a drug through skin by using a driving force and by rapidly disrupting the skin barrier. A well-known needleless injector, PowderJect® (PowderJect Pharmaceuticals, UK), shoots solid particles across the stratum corneum using high-pressure helium gas. Vaccines formulated in powder or particle forms can be delivered by this method. DNA vaccine coated on gold or tungsten particles has also been delivered into the epidermis using PowderJect® (Sloat et al. 2012b). Another needleless injector, Intraject®, uses nitrogen gas to drive liquid formulations across the skin (Tanner and Marks 2008). Along with PowderJect®, Intraject® has also been used to administer plasmid DNA for genetic immunization (Haensler et al. 1999; Manam et al. 2000; Mumper and Cui 2003; Aguiar et al. 2001; Chen et al. 2002, 2003, 2004). For more information about plasmid DNA immunization using jet injection devices, please refer to Sloat et al. (Sloat et al. 2012b ) and Chap. 32 in this volume (written by Y.C. Kim) .
28.2.2 Chemical Techniques
28.2.2.1 Chemical Penetration Enhancers
A molecule that enhances the permeation of drugs across the stratum corneum layer is called chemical penetration enhancer. Chemical penetration method provides certain advantages over the aforementioned physical methods, including design flexibility with formulation chemistry and easier prospect for a patch application (Prausnitz et al. 2004). Chemical enhancers partition into and interact with the stratum corneum to induce a temporary, reversible increase in the skin permeability (Shah et al. 1993). Chemical enhancers have many classes such as surfactants (e.g., Tween 20), fatty acids (e.g., oleic acid), terpenes (e.g., limonene), and solvents (e.g., ethanol) (Williams and Barry 2004). However, not all the chemical enhancers have been found to enhance the transdermal permeability significantly, particularly for high molecular weight molecules. Another major limitation of chemical enhancers is that they are often potent irritants to the skin (Prausnitz et al. 2004); and this safety issue further decreases the number of chemical enhancers that can be included in formulations (Subedi et al. 2010).
In our own previous studies, DNA application site on the skin is routinely hydrated with warm water or low concentration of sodium dodecyl sulfate (SDS) and cleaned with ethanol. Water, SDS, and ethanol can all potentially enhance skin permeation (Cui and Sloat 2006; Xiao et al. 2012; Yu et al. 2011). In a study by Heckert et al., it was demonstrated that combination of dimethyl sulfoxide (DMSO) with naked DNA applied topically onto unaltered skin of chicken generated a specific immune response that was stronger than that induced by conventional IM injection (Heckert et al. 2002).
28.2.3 Other Novel Techniques
Skin appendages such as hair follicles, sebaceous glands, and sweat glands have also been shown to play an important role in increasing skin permeability, particularly for large molecules. Appendages create a channel in the stratum corneum and facilitate the dermal absorption of topically applied compounds. Another method that has been researched extensively for enhancing skin permeability is the microneedle technology. Microneedles can disrupt the stratum corneum in the micron scale and thus can be used to enhance drug transportation into or across the skin. In this chapter, cutaneous DNA immunization by modifying the hair follicle cycle and by microneedle application is discussed in details.
28.3 Cutaneous DNA Immunization via the Hair Follicles
The structure and biology of hair follicles (HFs) make them a potential route for drug and vaccine penetration through the stratum corneum. The stratum corneum is hydrophobic in nature and thus resists large negatively charged DNA molecules from entering the skin. Data from several studies have demonstrated that the HFs are involved in the uptake and expression of plasmid DNA applied topically onto the skin, mostly by retaining the plasmid DNA and functioning as a reservoir. In a very early study in 1995, Li and Hoffman reported the targeting of a lacZ reporter gene-encoding plasmid to the HFs of mice using liposomes as a carrier (Li and Hoffman 1995). Their results demonstrated that the plasmid can be selectively targeted to the most important cells in the HFs. After topical application of the lacZ-encoding plasmid, expression was observed in hair matrix cells in hair follicle bulbs and in stem cells in the bulge area (Li and Hoffman 1995). A few years later, a study performed by Shi et al. showed that noninvasive gene delivery by pipetting adenoviruses or plasmid DNA that was complexed with liposomes onto mouse skin can produce a localized transgene expression, as well as systemic immune responses, against the protein encoded by the DNA (Shi et al. 1999). Moreover, it was shown that the production of even a small amount of protein in the skin is sufficient to generate a desired immune response (Shi et al. 1999). It was speculated that plasmid DNA applied topically may have penetrated into the skin via HFs, sweat ducts, or minor breaches on the skin surface (Shi et al. 1999).
28.3.1 Delivery Sites in the Hair Follicles
Hair follicular targets mainly include four sites: infundibulum, sebaceous gland, bulge region, and hair bulb (Knorr et al. 2009; Wosicka and Cal 2010). The infundibulum region creates the major entry point for topically applied substances, especially in the lower part where the epidermis is not fully differentiated. APCs, mast cells, and other immune cells are also located inside and around the infundibular epithelium, which makes HFs a potential target for cutaneous immunization. Sebaceous gland is another important therapeutic target site. It is associated with the etiology of androgenetic alopecia, acne, and other sebaceous gland dysfunctions; therefore, it can be used for the therapy of these diseases. The bulge region in HFs contains epithelial stem cells, which has a high proliferative capacity and multipotency. Thus, the bulge region is of particular interest for gene therapy targeting congenital hair disorders or genetic skin disorders. Finally, the hair bulb with the dermal papilla and the hair matrix connected to the blood vessels is important in the regulations of hair growth and pigmentation (Knorr et al. 2009; Wosicka and Cal 2010).
28.3.2 Hair Follicle Cycle
Along with HF morphology, transfollicular delivery also depends upon the functional status of HFs. Each HF goes through a highly regulated continuous cycle comprised of three major stages: anagen, catagen, and telogen (Paus and Cotsarelis 1999). In anagen (growth) phase, cells proliferate rapidly and continuously to form the inner root sheath and migrate upward to form the hair shaft. Catagen (regressing) phase is the end of mitosis where cell death of the lower follicle segment occurs. In telogen (resting) phase, the hair eventually shed. Under physiological conditions, 85–90 % of human scalp follicles are within the anagen phase (lasting for 2–6 years), 1–2 % of scalp follicles are in the catagen phase (lasting for 2–4 weeks), and about 10 % are in the telogen phase (lasting for 2–4 months) (Paus and Cotsarelis 1999).
Slominski et al. reported that the characteristics of the anagen phase include thickened dermal and epidermal layers of the skin, increased size of HFs, extension of follicles deep into the dermal adipose tissue, and initiation of melanin synthesis (Slominski et al. 1991). Otberg et al. found that the penetration of topically applied substances into the infundibulum is dependent on the active and inactive states of HFs. Active HFs exhibiting hair growth (anagen) and/or sebum production are open for the penetration process, but telogen follicles do not support any follicular penetration (Otberg et al. 2004).
Domashenko et al. showed that topical application of a lacZ gene-encoding plasmid complexed with liposomes (lipoplexes) led to efficient in vivo transfection of hair follicular cells, and the transfection was dependent upon the stage of the hair follicular cycle (Domashenko et al. 2000). Transfection occurred only at the onset of the growing stage of the hair follicular cycle created by hair depilation. Expression of β-galactoside gene was detected only in the HFs of mice transfected with lipoplexes that contained lacZ plasmid on the first, second, or third day after hair depilation. Transfection 3 days after the depilation did not result in any β-galactosidase activity. During the first 3 days after depilation, the HFs were in anagen-onset stage. Thus, the authors concluded that the timing of plasmid application when the HFs are just entering the anagen stage is critical for efficient in vivo transfection of HFs (Domashenko et al. 2000). Gupta et al. also supported this idea by reporting that gene transfection of HFs occurs only during the anagen-onset stage (Gupta et al. 2001). Thus, the anagen stage of HFs is important for transfollicular penetration.
Fan et al. demonstrated that topical application of plasmids encoding lacZ or hepatitis B surface antigen (HBsAg) to untreated mouse skin can induce specific immune responses (Fan et al. 1999). The authors also reported that topical gene transfer is dependent on the presence of normal HFs. Plasmid DNA was detected in and around the HFs in normal hair-bearing skin. The skin that lacks normal HFs failed to retain plasmid DNA. The authors further showed that normal HFs are important for the induction of immune responses. Application of plasmid DNA onto a skin area that lacks normal HFs in immunocompetent mice failed to generate an immune response against HBsAg (Fan et al. 1999).
28.3.3 Cutaneous DNA Immunization After “Cold” Waxing-Based Plucking
Anagen phase can be artificially induced by experimental methods including hair plucking, vigorous shaving, or chemical exposure such as caustic materials and depilatory agents.
Ishimatsu-Tsuji et al. defined mouse HF cycle by different gene expression patterns following hair plucking using wax (Ishimatsu-Tsuji et al. 2005). The period of up to 2 days after hair plucking (early anagen) is defined as the defense response phase, where mainly inflammation-related genes are upregulated. The period of 3–6 days following hair plucking (mid-anagen) is defined by keratinocyte proliferation processes, whereas late anagen and early catagen phase are characterized by multiple processes including keratin intermediate filament formation (Ishimatsu-Tsuji et al. 2005).
We hypothesized that topical application of plasmid DNA onto a skin area wherein the HFs are in the anagen-onset stage will induce a stronger immune response than when the HFs are in the telogen stage. In order to test this hypothesis, the HFs on the dorsal side of 6-week-old mice were induced (from telogen stage) into anagen-onset stage by hair plucking with a Veet® wax strip from Reckitt Benckiser, Parsippany, NJ, USA (i.e., “cold” waxing). Veet® wax strips come in the form of pre-waxed strips. Strip was placed over the hair-trimmed skin area and rapidly removed to detach the hair from the skin surface. Two days later, plasmid that encodes anthrax protective antigen (PA63) protein (pGPA) was applied onto the plucked skin area. Cholera toxin was used as an adjuvant. Our data showed that cutaneous immunization by applying the pGPA plasmid onto a skin area wherein the HFs had been induced into anagen-onset stage induced a stronger anti-PA antibody response, increased the antigen gene expression, and enhanced the retention of the plasmid DNA, as compared to topical application of the same pGPA plasmid onto a skin area wherein the HFs were left in the telogen stage (Shaker et al. 2007).
28.3.4 “Warm” Waxing Versus “Cold” Waxing-Based Hair Plucking
In the “cold” waxing-based studies, the hair plucking was performed by “cold” waxing, which in some cases can result in significant breakages of the hair at or below the surface of the skin. In order to remove the hair completely and expand the HF significantly, we used a “warm” waxing method to pluck hair, expecting that it would further enhance the penetration of plasmid DNA into the HFs and thus induce a stronger immune response than “cold” waxing-based hair plucking. In the “warm” waxing method, warm wax in viscous liquid form is directly applied onto a hairy skin area. The warmth of the wax is expected to allow the HF pores to expand and thus complete removal of the hair from the follicles becomes possible (Sloat et al. 2012a).
The immune responses induced by cutaneous immunization with the pGPA plasmid onto a skin area wherein the hair had been plucked 2 days earlier using “cold” versus “warm” waxing techniques were evaluated and compared. Plucking the hair by “warm” waxing resulted in an anti-PA IgG titer significantly higher than by “cold” waxing (Sloat et al. 2012a). Relatively more PA63 gene fragments were recovered from the skin area where the hair was plucked by “warm” waxing than by “cold” waxing, indicating that “warm” waxing led to more DNA uptake and/or retention in the skin than “cold” waxing (Sloat et al. 2012a).
Based on the studies performed in our lab, we conclude that the immune response induced by cutaneous DNA immunization via HFs can be enhanced by modifying the HFs or HFs cycle.
28.3.5 Follicular Vaccine Delivery Using Tape-Stripping Method
A new penetration-enhancing technique “cyanoacrylate skin surface stripping” (CSSS) was introduced by Schaefer and Lademann in 2001 and has been shown to be a promising method for transfollicular penetration by opening up the follicular infundibula (Schaefer and Lademann 2001). CSSS facilitates follicular penetration of vaccines by removing cellular debris and sebum from the hair follicular openings (Schaefer and Lademann 2001). Toll et al. used CSSS technique to deliver fluorescent microspheres of size 0.75–6.0 μm on human skin and demonstrated that without pretreatment with CSSS, the transfollicular microsphere penetration was below 25 % of the total number of HFs with a maximum penetration depth of 1500 μm in the hair follicles. However, CSSS pretreatment of the skin increased the transfollicular microsphere penetration to 35–87 % of the HFs with a penetration depth of more than 2300 μm (Toll et al. 2004).
Multiple efforts have also been made to explore nanoparticles as transfollicular carrier for vaccine delivery inside the skin. Vogt et al. investigated the penetration of 40, 750, and 1500 nm nanoparticles across human skin and their possible targeting to APCs inside the skin (Vogt et al. 2006). In their study, CSSS was performed prior to applying nanoparticles cutaneously. Flow cytometric data showed that after cutaneous application, only the 40 nm particles entered epidermal LCs, clearly indicating that only smaller nanoparticles can deeply penetrate into hair openings and through the follicular epithelium into perifollicular dermis. Thus, it was concluded that 40 nm nanoparticles, but not 750 or 1500 nm nanoparticles, are suitable for cutaneous immunization via the HFs (Vogt et al. 2006).
Mahe et al. performed a study to evaluate the potential of using the HF pathway for vaccine delivery. The authors studied the penetration of 40 or 200 nm fluorescent polystyrene nanoparticles as well as virus particles and demonstrated that both polystyrene nanoparticles and the modified vaccinia Ankara (MVA) viral particles penetrated deeply into HFs and were internalized by epidermal and dermal APCs. Topical application of ovalbumin-encoding plasmid DNA or MVA induced both humoral and cellular immune response. IgG response induced by ovalbumin-encoding plasmid DNA alone after cutaneous administration was found similar to that induced by ovalbumin protein delivered cutaneously in combination with cholera toxin adjuvant. However, in this study, authors preferred to perform tape stripping at the place of CSSS before applying vaccines onto the skin surface (Mahe et al. 2009).
In a clinical study performed by Vogt et al., it was demonstrated that cutaneous administration of influenza protein-based vaccine using CSSS technique promoted both CD4+ T and CD8+ T cell immune responses in human subjects, whereas intramuscular injection of the same vaccine generated only CD4+ T cell response (Vogt et al. 2008).
Another clinical study based on CSSS procedure was performed by Combadiere et al. (Combadiere et al. 2010). Immune response generated by cutaneous application of a combined tetanus and influenza vaccine suspension after CSSS procedure in the upper left arm area was compared with the intramuscular immunization with same vaccine. Results showed that cutaneous application of the vaccine was safe and that the inactivated influenza vaccine induced a CD8+ T cell response. Tetanus specific cellular response level was not detected (Combadiere et al. 2010).
28.4 Microneedle-Mediated Cutaneous DNA Immunization
28.4.1 Microneedles: A Novel Approach for Enhancing Cutaneous Permeation
Microneedles are one of the new technologies studied in transdermal drug delivery. This is a simple, pain-free, and minimally invasive technology that helps the delivery of large molecular weight and hydrophilic compounds across skin. Microneedles consist of a plurality of microprojections of different shapes, sizes, and heights, which are attached to a base support. Application of microneedles onto skin surface creates micron size transport pathways, which allow the permeation of macromolecules inside the skin. According to Kaushik et al., microneedles perforate the stratum corneum, avoiding any contact with nerve fibers and blood vessels present in the dermis layer (Kaushik et al. 2001). Therefore, the major benefit of using microneedles is the pain-free delivery of both small and large molecular weight compounds (Kaushik et al. 2001; Prausnitz 2004).
The concept of microneedle first came in the 1970s; however, technological limitations at that time prevented the product concept from being executed (Gerstel and Place 1976). In the 1990s with the beginning of high-precision microelectronics industrial tools, microneedle manufacturing also became a reality. The first microneedle array reported in the literature was made from silicon wafer and was developed for gene delivery (Hashmi et al. 1995). In that study, the needles were inserted into nematodes to increase molecular uptake and gene transfection. After transfection, the gene of interest was expressed in the progeny of the injected worms (Hashmi et al. 1995). The first paper to report microneedles for transdermal drug delivery was published by Henry et al. in 1998, who demonstrated that microneedles can be used to increase skin permeability (Henry et al. 1998). An array of solid microneedles of 150 μm length was used to create micropores in human epidermis. These micropores increase the skin permeability by three orders of magnitude for a small model compound calcein. The transportation of calcein took place through the leakage pathways created between the needles and the skin. When the needles were removed from the skin, the skin permeability was increased by another order of magnitude (Henry et al. 1998). In a follow-up study, McAllister et al. studied the permeability of various compounds across cadaver skin using Franz diffusion cell and noted that insulin, bovine serum albumin, and latex nanoparticles (up to 100 nm size) could cross the skin pretreated with microneedles (McAllister et al. 2003). These early studies demonstrated that skin permeability can be increased using microneedles.
Microneedles are somewhat like traditional needles but are fabricated on the micron scale. The most commonly used fabrication materials are metals, silicon, silicon dioxide, polymers, and glass. The first few microneedle devices were fabricated from silicon (Henry et al. 1998; Hashmi et al. 1995). Later, other materials such as stainless steel (Verbaan et al. 2007), dextrin (Ito et al. 2006a, b), glass (Wang et al. 2000), ceramic (Ovsianikov et al. 2007), maltose (Kolli and Banga 2008), galactose (Donnelly et al. 2009), and various polymers (McAllister et al. 2003; Park et al. 2005) have also been used to fabricate microneedles. The most commonly used methods for the manufacturing of microneedles are chemical isotropic etching, injection molding, reactive ion etching, surface/bulk micromachining, polysilicon micromolding, lithography-electroforming-replication, and laser drilling (Park et al. 2005; Trichur et al. 2002; Yang and Zahn 2004; Davis et al. 2005; Stoeber and Liepmann 2005). The two basic designs of microneedles are in plane and out plane designs.
28.4.2 Methods of Using Microneedles to Administer Vaccine
The use of microneedles in delivering vaccines into the epidermis and dermis compartments of skin is a very attractive approach for cutaneous vaccination. Four different microneedle designs have been developed for the delivery of vaccines inside the skin.
28.4.2.1 Solid Microneedles
The simplest method of cutaneous immunization using microneedles is to create pores on the skin with a solid microneedle array and then apply vaccine onto the perforated skin surface. By piercing the skin, the permeation of vaccine can be increased by manyfolds. This method has been used to deliver proteins (e.g., bovine serum albumin) and genetic materials (e.g., plasmid DNA) in vitro and in vivo into the skin (Chabri et al. 2004; Coulman et al. 2006; Henry et al. 1998; Lin et al. 2001; Martanto et al. 2004; Park et al. 2005; Pearton et al. 2008). For example, Ding et al. demonstrated a major improvement in the immunogenicity of diphtheria toxoid in a mouse model when the antigen was applied onto a skin area pretreated with microneedles (Ding et al. 2009).
28.4.2.2 Coated Microneedles
Solid microneedles can also be coated with a vaccine. Coating is usually applied by dipping microneedles in a vaccine formulation. Coated microneedles can be inserted into skin and quickly removed after a few seconds, leaving the vaccine inside the skin. Using this approach, proteins, DNA, and viral particles such as influenza virus particle have been delivered to the skin in vitro and in vivo (Gill and Prausnitz 2007a, b; Hooper et al. 2007; Matriano et al. 2002; Widera et al. 2006; Xie et al. 2005; Zhu et al. 2009; Gill et al. 2010). Matriano et al. demonstrated that cutaneous immunization with 300-μm long titanium microneedles coated with 1 μg of ovalbumin generated a 100-fold increase in immune responses, compared to intramuscular injection of the same dose (Matriano et al. 2002). In another study, Widera et al. found that the immune responses induced by cutaneous immunization with microneedles coated with ovalbumin are dose dependent; however, the immune responses were independent of the depth of delivery, the density of microneedles, or the area of application (Widera et al. 2006).
28.4.2.3 Hollow Microneedles
One hollow microneedle or an array of hollow microneedles can be used to deliver liquid vaccine formulations inside the skin. Hollow microneedles allow a controlled quantity of antigens to be delivered inside the skin with a definite rate. Hollow microneedles have been used to deliver influenza and anthrax vaccines in animal models (Alarcon et al. 2007; Mikszta et al. 2005, 2006). Van Damme et al. delivered α-RIX influenza vaccine (3.3 μg of hemagglutinin per strain) in human volunteers using 450-μm long hollow microneedles and reported that the immune response induced was similar to that induced by 15 μg hemagglutinin per strain delivered intramuscularly (Van Damme et al. 2009).
28.4.2.4 Dissolvable Microneedles
Microneedles can also be prepared from polymers or saccharides with vaccine encapsulated inside. After inserting inside the skin, microneedles and vaccine dissolve within few minutes, and the backing layer is discarded. Sullivan et al. reported that inactivated influenza virus (A/PR/8/34) encapsulated in polyvinylpyrrolidone dissolvable microneedles induced strong humoral and cellular immune responses and also provided a defense again influenza challenge (Sullivan et al. 2010).
28.4.3 Microneedle-Mediated Cutaneous Immunization
As discussed earlier, it is a well-known fact that skin offers a great immunologic environment compared to muscle and subcutaneous tissues. However, so far there is no simple, reliable, and safe method available to deliver vaccines into the skin efficiently in a cost-effective way to a large population of people. The microneedle device has the potential to address these problems to some extent. The painless microneedles not only can improve patient compliance but also enable the targeting of antigens to the immune cell-rich skin layers. In the past decades, many different vaccines and/or antigens, including live attenuated microorganisms, proteins, and plasmid DNA, have been delivered into the skin using microneedles. For example, influenza vaccination using whole inactivated influenza viruses coated on microneedles has been studied extensively. Studies have shown a complete protection against infection after microneedle-mediated cutaneous immunization with influenza virus A/Puerto Rico/8/34 (H1N1), influenza virus A/California/04/09 (H1N1), and influenza virus A/Aichi/2/68 (H3N2) (Zhu et al. 2009; Koutsonanos et al. 2009, 2011). Along with whole inactivated influenza virus, virus like particles (VLP) coated on microneedles were also studied. It was shown that cutaneous immunization with avian H5 influenza VLPs coated on stainless steel microneedles induced a stronger immune response in a mouse model, relative to intramuscular injection (Song et al. 2010a, b). Microneedles have also been used for the delivery of recombinant subunit vaccines, such as trimeric influenza hemagglutinin protein. Upon administration, protein antigen coated on microneedles induced an enhanced immunity, relative to the subcutaneous injection of the same protein antigen in mice (Weldon et al. 2011). In a study in our laboratory, we have demonstrated that ovalbumin chemically conjugated onto solid lipid nanoparticles and then administered cutaneously onto a skin area pretreated with microneedles induced a stronger immune response as compared to cutaneous immunization using ovalbumin alone (Kumar et al. 2011). The dose of the antigen determined whether the microneedle-mediated immunization can induce a stronger immune response than subcutaneous injection of the same antigen conjugated on the solid lipid nanoparticles (Kumar et al. 2011). Finally, plasmid DNA has also been explored extensively for microneedle-mediated cutaneous immunization, which is discussed below in details.
28.4.4 Microneedle-Mediated Cutaneous DNA Immunization
Cutaneous DNA immunization has been performed using gene gun and electroporation techniques and was found to improve the immune responses by manyfolds in human and nonhuman primates (Donnelly et al. 2003). However, gene gun and electroporation techniques are tedious and require a vaccination protocol and equipment (Nicolas and Guy 2008). Microneedles as a new technology may deliver DNA vaccine painlessly into the skin and is potentially suitable for inexpensive mass production. The first study showing the delivery of DNA using microneedles was performed by Hashmi et al. in 1995. In their study, DNA-coated microprobes were injected in the cuticle of nematode Heterorhabditis bacteriophora to express a foreign gene, β-galactosidase (Hashmi et al. 1995). After this study, microneedle devices were shown to increase the skin permeability of plasmid DNA in vitro (McAllister et al. 2000). However, there was not any systematic in vivo study performed until 2002, when Mikszta et al. reported the first in vivo study using microneedles to deliver DNA vaccine topically into the skin. In that study, microenhancer arrays (MEAs, i.e., microneedles) were fabricated using potassium hydroxide etch technique. The array was fabricated on 1 cm2 microchips and had the projection height ranging from 50 to 200 μm. Naked plasmid DNA encoding firefly luciferase was administered to mice topically using the MEAs. The arrays were dipped into plasmid DNA solution and scraped multiple times across the skin of mice to create microabrasions. The results demonstrated that the mean luciferase activity in groups treated with six or more passes of MEA were 1000- to 2800-fold higher in comparison to topical controls. Untreated skin was used as a control for possible delivery through HFs. The gene expression level in the MEA-treated groups was also found similar or greater than after intramuscular and intradermal injection of plasmids. The results showed that even a single pass of the MEA across the skin enabled gene transfer. The authors also used a plasmid DNA that encodes hepatitis B surface antigen and reported that MEA-based cutaneous immunization induced a stronger immune response with less variability after the second and third immunizations, as compared to immunization by hypodermic needle injection. Both hypodermic needle injection and MEA-based delivery induced similar antigen-specific cytotoxic T lymphocyte (CTL) responses (Mikszta et al. 2002). The significance of this study is that it demonstrates the feasibility of cutaneous DNA vaccination using microneedles.
In separate studies by Birchall et al. and Coulman et al., it has been demonstrated that microneedles can facilitate the delivery of plasmid DNA into the epidermis layer of the human skin, and the genes encoded by the plasmid can be expressed in the skin. Birchall et al. delivered pCMV-β plasmid into the skin with the help of silicon microneedles and demonstrated a detectable gene expression in the microchannels. In control groups, where DNA was delivered without microneedle treatment, expression was not observed. Coulman et al. also used silicon microneedle arrays to create micropores through the stratum corneum of human skin samples to test the ability of the conduits to facilitate the delivery of pCMV-β plasmid. Their results demonstrated that these micropores facilitated the delivery of plasmid DNA, and gene expression study confirmed that naked plasmid DNA was able to be expressed in excised human skin. However, the presence of limited conduits that were positive for gene expression indicated that there is a need to optimize the microneedle device morphology, the application method, and the DNA formulation in order to obtain a higher gene expression (Birchall et al. 2005; Coulman et al. 2006).
Alarcon et al. performed an animal study to use microneedles to deliver three different types of influenza vaccines: whole inactivated influenza virus, trivalent split-virion human vaccine, and plasmid DNA encoding the influenza virus hemagglutinin. In their study, rats were chosen as an animal model since rat skin is thicker than the total length of microneedles (i.e., 1 mm) used in the study. Antigens were administered intradermally using hollow microneedles and intramuscularly using hypodermic needles. Results showed that intradermal delivery using hollow microneedles generated an antibody response similar to intramuscular injection; however, dose-sparing effect was achieved when microneedles were used (Alarcon et al. 2007).
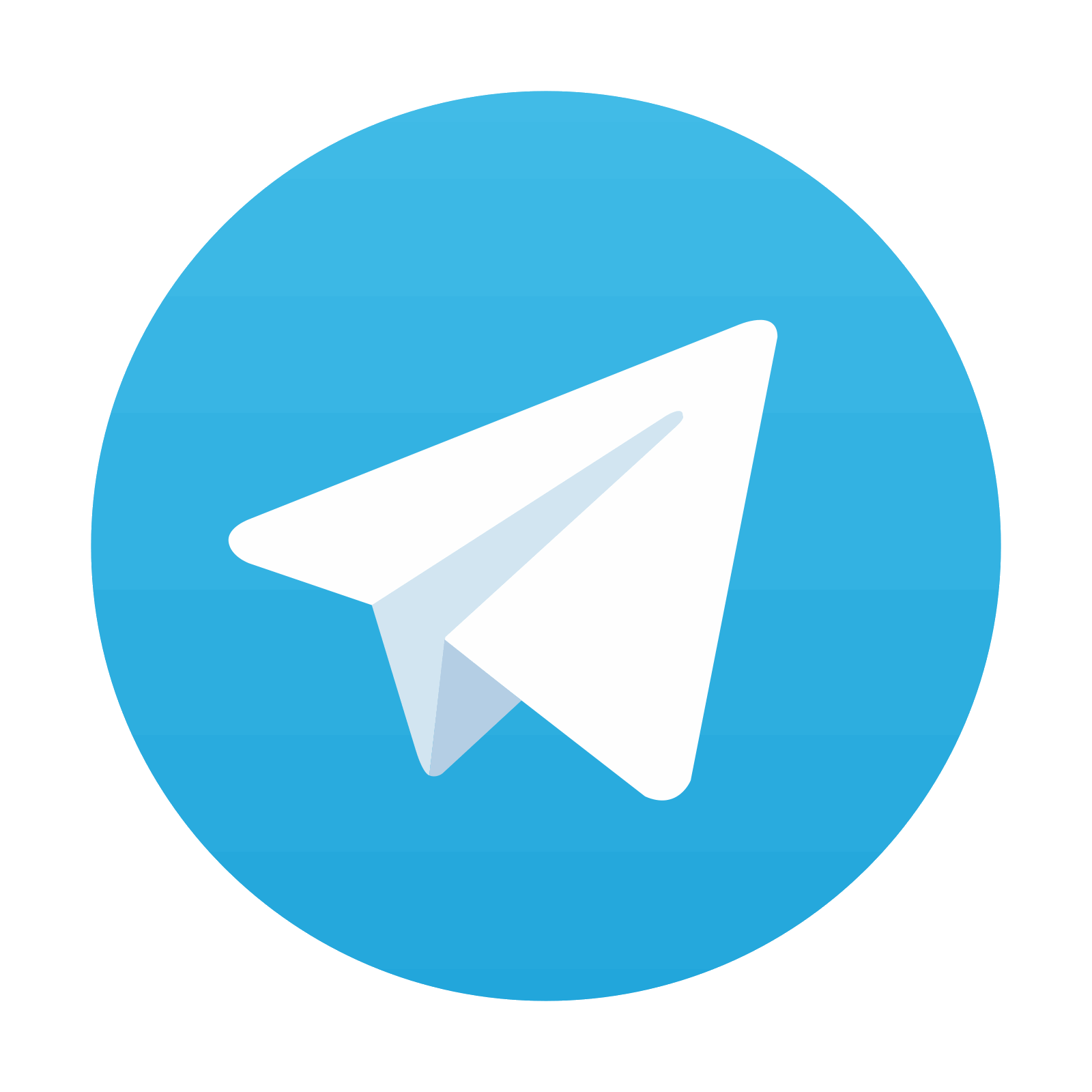
Stay updated, free articles. Join our Telegram channel
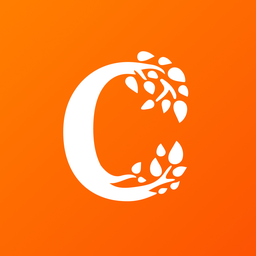
Full access? Get Clinical Tree
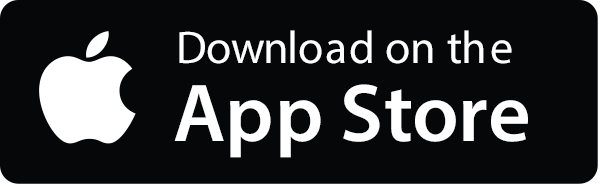
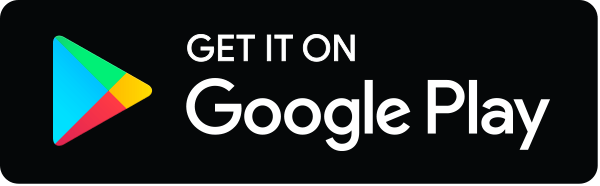