Fig. 14.1
Schematic representation of possible mechanisms of tolerance in reconstructive transplantation. BM bone marrow, Tregs T regulatory cell
In the attempt to translate these protocols to the clinic, multiple large animal studies have been conducted in the past decade. In fact, protocols that are feasible in mice and small animals often do not yield the same results in large animal models and humans due to several biological differences that affect both metabolic responses to drugs and the intrinsic–innate and adaptive immunologic responses [75, 77] .
The first clinical evidence that cellular strategies may promote tolerance induction was prompted by the observation that patients undergoing myeloablative therapy combined with both solid-organ transplantation and allogeneic bone marrow transplantation (BMT) became tolerant of their kidney allograft [18]. The repopulation of host myeloid lineages with donor hematopoietic cells induced tolerance toward the donor kidney graft, highlighting the potential role of cell-based approaches for establishing tolerance in VCA. Since then, numerous studies have investigated the use of different cell types as possible immunomodulators. These cell populations include hematopoietic stem cells (HSCs), Tregs, dendritic cells (DCs), and mesenchymal stem cells (MSCs) among others.
Mixed Chimerism
Chimerism is defined as the coexistence in the host of donor and recipient hematopoietic cells. The use of myeloablative conditioning regimens leads to the establishment of a condition termed full chimerism, in which the complete replacement of the recipient’s bone marrow with donor cells occurs. Severe morbidity is associated with the establishment of full chimerism, largely due to the toxicity of myeloablative regimens that expose the patient at risk for severe infections and GvHD. For this reason, this procedure was historically reserved to patients receiving organs from human leukocyte antigen (HLA)-matched siblings. More recently, Sykes et al. have shown that mixed chimerism can be induced in adult recipients of HLA-mismatched BMT by a nonmyeloablative conditioning regimen, allowing for wider application of such protocols in patients receiving an organ from unrelated donors [94].
The preliminary studies to achieve these goals were possible due to the development of the Massachusetts General Hospital (MGH) miniature swine as a model for translational research in transplantation [76]. These animals were bred to fix the MHC antigens (while maintaining variability for minor antigens). This allowed selection of a variety of MHC mismatches including but not limited to:
1.
Syngeneic (complete match of both alleles for MHC class I and II in both donor and recipient swine leukocyte antigens (SLA) , i.e. SLAaa→SLAaa)
2.
Single-haplotype mismatch (sharing of only one allele of class I and II, i.e., SLAac→SLAad)
3.
Full mismatch (no sharing of any haplotype of class I and II between donor and recipient, i.e., SLAcc→SLAdd) clinical scenarios
For this reason, the MGH miniature swine model allowed the investigation of different conditioning regimens for tolerance induction of organs and testing of such protocols, while simulating different genetic disparity, such as those present in HLA-identical siblings (full MHC match, minor antigens mismatch only), parents to offsprings, and single haplotype-mismatched siblings (single haplotype MHC mismatch) or nonmatched living-related or cadaveric-donor transplants (full MHC mismatch) [73, 76].
Most of the preliminary studies aimed at the treatment of hematologic malignancies by means of mixed chimerism were conducted in the MGH miniature swine and utilized leukapheresis to mobilize large quantities of HSCs from peripheral blood [14, 34]. Such protocols, however, are not readily translatable to the scenario of clinical reconstructive transplantation, as drug-induced mobilization of HSCs and leukapheresis is not feasible in the deceased donor. Clinically, translational protocols for VCA, indeed, require the use of induction therapies that can be administered in the immediate pre/perioperative time period.
Currently, mixed chimerism has been the only approach to successfully achieve tolerance in solid-organ transplantation in nonhuman primates (NHPs), despite numerous protocols capable of inducing tolerance in other animal models including rodents and swine [16, 93]. Based on promising experimental results, clinical trials have been developed to study combined BMT and kidney transplants first in MHC-matched patients affected by multiple myeloma-induced renal failure and, subsequently, in kidney transplants in MHC-mismatch recipients with no malignancy [47, 90]. Importantly, recipients of these cell-based tolerance protocols achieved long-term tolerance to their allografts, with the longest immunosuppression-free survival exceeding 10 years. Furthermore, no evidence of chronic rejection has been observed and a lower-than-expected incidence of GvHD has been reported following these protocols. (We will defer an in-depth discussion of mixed chimerism-based approaches to Chap. 16.)
T Regulatory Cells
Tregs, previously known as T suppressor cells, play a crucial role in maintaining tolerance to self-antigens and balancing immune responses that otherwise would be harmful to the host. In fact, Treg dysfunction has been implicated in a variety of autoimmune and inflammatory diseases in humans. Tregs can be classified as either thymus-derived naturally occurring (CD4+/CD25hi/FOXP3+) or peripherally induced (FOXP3) and upregulated in response to antigen exposure [81]. FOXP3 is a master transcription factor that regulates Treg activation and IL-2, IL-10, and transforming growth factor β (TGF-β) pathways appear essential in controlling Treg interactions with other cells .
In transplantation, Tregs are thought to be highly important in maintaining immune suppression and preventing acute and chronic graft rejection. Experimental transplantation models that target Treg function have been highly promising and often utilize either in vivo or ex vivo techniques to activate this cell population. In vivo strategies involve antigen exposure to induce expansion of Treg populations, resulting in prevention of allograft rejection in multiple animal models (Issa and Wood 2012). Co-stimulatory blockade—e.g., targeting cluster of differentiation (CD)28, CTLA4, or CD40L)—or lymphocyte depletion protocols (monoclonal antibodies, cytotoxic agents, radiation) have also shown success in activating Tregs and promoting graft tolerance [71, 100].
Ex vivo strategies that employ Tregs have utilized blood obtained from the peripheral circulation or umbilical cord. However, isolation of these cells is predicated on being able to efficiently identify characteristic surface markers. Given the nonspecific nature of CD25 and FOXP3 expression, other markers have been described to isolate Tregs, including CD44, CD45RA, CD49b, CD69, CD127, CD152, and latency-associated peptide (LAP). For example, Tregs with low expression of CD127 have been demonstrated to suppress vessel allograft and skin graft rejection in humanized mice models [39]. Additionally, Tregs expressing LAP may have superior in vivo immunosuppressive capabilities mediated via TGF-β signaling. This subset of Tregs was shown to successfully block experimental autoimmune encephalomyelitis in mice [13].
Ex vivo expansion of Tregs has been described using αCD3/αCD28 microbeads and recombinant human interleukin (IL)-2 [78]. Selection of human Tregs based on CD69 and CD71 expression was utilized to reduce immune-mediated injury to human skin grafts in a mouse model, suggesting that anti-donor Tregs can be “customized” for clinical transplantation protocols [80]. Recently, scalable techniques have been described to harvest and efficiently expand Treg populations from peripheral blood using artificial antigen-presenting cells (APCs) (loaded with anti-CD3 antibody) expressing the high-affinity fragment crystallizable (Fc) receptor and CD86 [32]. These populations were infused into mice and markedly reduced GvHD lethality. Collectively, these studies demonstrate the potential of Treg modulation to maintain transplant graft tolerance .
Dendritic Cells
DCs are bone marrow-derived APCs that play a critical role in modulating the immune response. Although comprising less than 1 % of circulating blood mononuclear cells, they are found throughout the body and appear to regulate T cell physiology. Multiple subsets of DCs have been characterized based on distinct phenotypic and functional properties. Although initially described as key initiators of the adaptive immune response, they are now recognized as sentinel regulators of both innate and adaptive immune responses [62].
The two broadest categories of DCs include classical DCs and plasmacytoid DCs. Classical DCs are largely involved in antigen presentation and T cell activation, whereas plasmacytoid DCs function to secrete interferons and other cytokines [91]. Importantly, both classes of DCs do not directly engage in effector activities. Basic science studies on DC function in mice have established surface marker profiles (e.g., CD8+/CD103+ and CD11c+) to characterize key functional properties but likely underestimate the significant heterogeneity in DC function [21, 28]. Much less is known about human DCs but genomic analyses demonstrate substantial conservation between mice and human DCs [17]. Recently, heterogeneous DC subpopulations that express Blood Dendritic Cell Antigen-3 (BDCA-3) have been identified in human blood, lymph nodes, and spleen and may correlate with established murine markers. However, much work remains in identifying and characterizing DC subsets in humans.
Given the primary role of DCs in regulating the immune response, recent transplant immunology research has focused on targeting subpopulations of DCs that may be responsible for promoting and maintaining tolerance [69]. In a mouse cardiac allograft model, immature bone marrow-derived DCs were able to significantly prolong graft survival compared to mature DCs [66]. In a mouse study on allogeneic BMT, a subset of DCs displaying high levels of MHC and low levels of co-stimulatory markers induced protective effects against GVHD, demonstrating the therapeutic potential of DCs [83].
Researchers have also demonstrated the ability to augment the tolerogenic effects of DCs via manipulation of co-stimulation pathways. For example, modulation of the CD40 pathway regulates DC–T cell interactions and promotes cardiac allograft survival in mice [65]. Additionally, blockade of the CD80/CD86–CD28 co-stimulatory pathway has been shown to prolong survival of hepatic allografts in a mouse model 63]. In a different mouse cardiac allograft model, preoperatively infused DC precursors treated with immunomodulatory signals have been demonstrated to extend allograft survival [1]. Most recently, DCs treated with the co-stimulation blocking agent CTLA4Ig were infused preoperatively in a NHP kidney allograft model, resulting in significantly prolonged graft survival [22] . These studies and others highlight the therapeutic promise of DC-based approaches for composite tissue transplantation.
Donor Hematopoietic Cells
Multiple approaches for tolerance induction have been developed that employ donor BMT [87]. Donor hematopoietic cells reach the recipient thymus, resulting in the negative selection of donor-reactive T cells and theoretically limiting the host response to donor antigens. Several groups have demonstrated improved chimerism using intraosseous delivery of bone marrow cells compared to standard intravenous delivery in a rat model [49]. Additionally, greater numbers of donor cells were detected in thymic and lymphoid tissues after intraosseous transplantation compared to intravenous techniques [49]. Enhanced chimerism and hind-limb transplant survival was also demonstrated in rats when intraosseous delivery of HSC was employed compared to no HSC therapy [88].
The combination of nonmyeloablative regimens with donor bone marrow cell delivery may be a viable strategy for tolerance induction in composite tissue allotransplantation. In a rat osteomyocutaneous flap model, recipient rats were pretreated with TBI , transplantation of selectively depleted T cells (lacking αβ-T cells), anti-lymphocyte serum, and short-course tacrolimus to generate mixed allogeneic chimeras [74]. Although peripheral blood chimerism was lost by 6 months, the donor bone compartment maintained long-term chimerism that appeared to sustain tolerance to the allograft.
Successful combined bone marrow and kidney transplantation from HLA single-haplotype-mismatched living-related donors has demonstrated the clinical potential of nonmyeloablative conditioning protocols for tolerance induction [46]. Importantly, these clinical trials highlight the relevance of transplanted hematopoietic cells in promoting immune tolerance . In addition to pretransplantation strategies, posttransplantation infusion of HSCs has also been used successfully, most notably in the first partial human face transplant [20]. Ongoing studies are examining the role of donor HSC infusions as maintenance immunosuppression following hand transplantation. Taken together, these studies suggest that hematopoietic-based strategies may be a key component of future tolerance induction protocols.
Facilitating Cells
Facilitating cells are a rare population of bone marrow-derived cells that enable allogeneic stem cell engraftment. Extensive studies have demonstrated that facilitating cells are neither mature T, B, or natural killer (NK) cells [15]. They exhibit lower levels of CD8 expression and lack the T cell antigen receptor (TCR) phenotype characteristic of T cells [42]. Studies have suggested that these cells are related to precursor plasmacytoid DCs and may have a similar cytokine activation profile [3, 25].
Experimental models of BMT have demonstrated that facilitating cells promote stem cell engraftment, inhibit GvHD, and induce tolerance [15]. Researchers have even shown that facilitating cells can sustain the engraftment of both adult and fetal stem cells in animal models of BMT [26]. Although the utility of these cells in solid-organ transplantation has not been extensively explored, facilitating cells represent a promising approach to establish allograft tolerance, while avoiding myeloablation and minimizing the risk of GvHD [40]. More recently, Leventhal et al. used mobilized cells enriched for HSCs and graft-facilitating cells combined with a nonmyeloablative conditioning in kidney transplant recipients across an MHC mismatch (nonrelated donor/recipient pair) [61]. In this phase 2 clinical trial, five of the eight kidney recipients displayed durable chimerism and were rendered immunosuppression-free 1 year after transplantation. No signs of GvHD or engraftment syndrome (a constellation of symptoms and signs following HSC transplantation and that include fever, erythrodermatous skin rash, and noncardiogenic pulmonary edema) [89] were observed in these patients. Testing this encouraging protocol in a larger patient cohort would provide further data to potentially allow its widespread use in the clinic.
Mesenchymal/Adipose-Derived Stem Cells
MSCs are a multipotent population of progenitor cells that have been described in many human tissues. Although originally identified in bone marrow, mesenchymal-like stem cells have also been found in adipose tissue (the adipose-derived stem cell—ASC) but their true identity and physiologic relevance remain unclear [59, 60]. In vitro, these cells can be directed to differentiate into several mesenchymal lineage cells, including fat, bone, and cartilage. They have also been shown to exhibit potent immunoregulatory properties [103] and have been increasingly explored as therapeutic cells for a wide variety of diseases [55].
The immunosuppressive effects of MSCs have been studied in various allotransplantation models in mice. For example, allogeneic islet graft take was markedly improved by therapeutic MSCs via mechanisms linked to matrix metalloproteinases and T cell expression of CD25 [19]. MSCs have also been used with rapamycin to augment cardiac allograft survival [27]. Interestingly, labeled MSCs could be tracked to lymphoid organs and cardiac grafts in tolerant recipients. In a kidney-allotransplant model, the delivery of MSCs pretransplantation promoted Treg pathways and graft survival, whereas posttransplantation delivery of MSCs resulted in early graft rejection [11]. The role of MSCs in VCA remains unclear, but the potent paracrine mechanisms observed in wound regeneration models suggest that MSCs may be a valuable tool to induce tolerance [2].
In this regard, MSCs have also been studied in large animal models of vascularized composite tissue transplantation. In a swine hind-limb allotransplantation model, MSC delivery was shown to prolong allograft survival and the combined use of MSCs, BMT, and cyclosporine A demonstrated the greatest degree of survival with no signs of GvHD and the lowest levels of rejection [52]. Similarly, a swine hemi-face allotransplantation model demonstrated improved graft survival with combined MSC infusion and cyclosporine A [54]. These findings were associated with increased markers for regulatory T cells and suppressed inflammatory signaling.
Researchers have also studied the immunomodulatory effects of intravenous ASC delivery following hind-limb transplantation in a rat model [53]. Allografts were shown to persist longer with ASC infusion via mechanisms linked to blockade of inflammatory cytokines and regulation of T cell function. ASCs have even been shown to augment engraftment of stem cells and to prevent GvHD in vivo [107]. Given their ease of harvest, relative abundance in Westernized societies, and the growing obesity epidemic worldwide, ASCs have tremendous translational potential as a therapeutic cell type [64].
Other Regulatory Cell Populations
Regulatory immune cells, including the previously discussed regulatory T cells, play a critical role in modulating the host immune response and can dictate long-term outcomes after allotransplantation. The broad classification of immune cells as T cells, B cells, macrophages, etc., grossly oversimplifies the complexity of these important cell populations. Recent immunology research has shed light on several cell subpopulations that may facilitate cell-based approaches to induce allograft tolerance [37, 38]. These cell subsets and others have been utilized in clinical studies and shown to improve graft tolerance. We refer the reader to an excellent recent review on this topic [2012].
Studies in renal transplant recipients that no longer require immunosuppression have demonstrated greater numbers of B cells and increased expression of B cell-related genes [79]. Regulatory B cells are a rare, immature subpopulation of B cells that have been described in both mice and humans (CD19+/CD24hi/CD38hi). A major property of these cells is their propensity to secrete IL-10, a potent immunomodulatory cytokine that controls T cell differentiation [23]. Another important signaling pathway appears to be CD40-associated activation of IL-10 and induction of Treg cells. Although B-cell-activated T cells appear more potent than plasmacytoid DC-activated T cells, the relevance to human allotransplantation remains unclear [109]. Studies in kidney transplant recipients suggest that regulatory B cells may facilitate the appearance of Treg cells later after induction therapy, suggesting that therapeutic expansion of regulatory B cells may be a viable strategy in the clinical setting [30].
Regulatory macrophages play a diverse role in tissue repair and immune regulation. Although this cell population remains ill-defined, these cells secrete IL-10 and can develop a suppressive phenotype upon interaction with Treg and B cells [95, 104]. In mice, blockade of macrophage populations in recipient animals worsened GvHD and resulted in higher numbers of donor T cells in a HSC transplantation model, indicating that macrophages may have a protective role in transplantation [29]. In promising early studies, kidney transplant recipients that received regulatory macrophage infusions required reduced levels of tacrolimus and maintained good early graft function [35]. These studies and others suggest that cell-based approaches to regulate the immunologic response after allotransplantation may be an effective strategy to promote graft tolerance.
Tolerance Protocols in VCA
Most of the research on allograft tolerance over the past few decades has focused on solid organs. However, recent research has increasingly focused on VCA. The presence of skin, the most immunogenic of tissues, in most VCA cases makes the translation of these protocols from solid-organ transplantation to VCA extremely challenging. In these protocols, heavy immunosuppressive therapy has been necessary to avoid rejection of these tissues. In this section, we discuss the regimens utilized in large animal models (swine and NHPs) and humans.
Swine
Several groups have utilized the MGH miniature swine models to study tolerance induction in VCA using different protocols based on mixed chimerism and various surgical models [51, 59, 98]. Furthermore, swine, canine, and NHP models have been used to investigate diverse strategies to reduce the number and dose of immunosuppressive drugs [5, 6, 12, 68].
The first attempts to achieve tolerance in VCA were performed using heterotopic limb transplants in the swine model. These experiments entailed the use of an osteomyocutaneous composite graft that included the distal part of the femur, the entire knee joint, and parts of tibia and fibula together with the surrounding muscles. The donor hind-limb allograft was transplanted to a subcutaneous abdominal wall pocket in the recipient animals. In contrast with the controls, which rejected the allografts in approximately 12 weeks, experimental animals receiving a calcineurin inhibitor (cyclosporine A) displayed long-term graft survival for up to 47 weeks following transplantations [57]. The presence of a bone marrow component in the donor graft-induced transient mixed microchimerism in the peripheral blood, assessed by the presence of pig allelic antigen positive (PAA+) donor cells in recipients lacking such antigens (PAA-). Interestingly, despite loss of chimerism in this model, the experimental animals remained tolerant of their allografts [9]. However, a skin component was not present in this model and additional immunomodulation , by means of BMT, to assess the validity of this model across disparate mismatch barriers was not attempted in these early studies. When a skin paddle was added to the design of the graft, further studies on MHC-matched/minor antigens mismatch models elucidated the concept of “split tolerance,” according to which the simultaneous acceptance of some of the graft tissues (i.e., muscle and bone) and the rejection of a more immunogenic tissue, such as skin, was possible due to the high immunogenicity of the skin component [67].
In the scenario of clinical reconstructive transplantation, however, it seems unlikely to find a fully matched donor/recipient couple to which such regimens would apply. For this reason, subsequent studies have explored clinically relevant models involving transplantation of vascularized composite allografts across partial and full MHC mismatches. In one of these studies, following T cell depletion, cytokine-mobilized peripheral blood mononuclear cells (CM-PBMCs) or bone marrow cells were transplanted at the time of VCA together with a short course of a calcineurin inhibitor. Donor-specific unresponsiveness and tolerance to the musculoskeletal components of the grafts were observed in both the CM-PBMC and the BMT recipients. Similar to the previous studies, the skin component was not accepted in both models, and peripheral chimerism was observed only in the group receiving CM-PBMCs. However, skin GvHD was observed in all the animals that displayed macrochimerism, making this protocol suboptimal for translation in the clinic [31].
Tolerance to skin in VCA across a single-haplotype MHC mismatch in MGH miniature swine was first reported by Horner et al. Donors were mobilized using stem cell factor and IL-3 and then leukapheresed to collect the donor hematopoietic product, which was then infused to recipients conditioned with 100 cGy TBI, T cell depletion and a 45-day course of cyclosporine A. Using this protocol, one animal achieved long-term multilineage mixed chimerism in peripheral blood and tissue chimerism in the thymus and in the bone marrow without developing GvHD [33]. More recently, the establishment of multilineage mixed chimerism across MHC haploidentical donor/recipient combinations was obtained using a conditioning regimen with CD3 immunotoxin (pCD3-DT390), TBI, hematopoietic cell transplantation and a 45-day course of cyclosporine A, leading to long-term survival of VCAs in this model [58]. As these studies further support the evidence that stable mixed chimerism can induce indefinite survival of a VCA, migration toward more clinically applicable protocols that do not require cell mobilization and donor treatment before transplant is paramount, since the possibility of conditioning a patient in the setting of clinical VCA is unlikely. The multilineage chimerism and bone marrow engraftment is thought to provide constant antigenic stimulation, thereby inducing central tolerance by prompting negative selection of donor-reactive thymocytes.
Furthermore, it has been hypothesized that peripheral regulatory mechanisms by T regulatory cells may allow for peripheral tolerance induction by deletion of donor-reactive clones escaped from the thymus [106].
More recently, the consideration that vascularized bone marrow may not be present in every type of VCA has led to the design of a reliable VCA model lacking a bone segment. This myocutaneous graft is harvested from the pig groin (based on the saphenous and femoral vessels) and reanastomosed to the common carotid artery and internal jugular vein in the cervical region [59]. This model allows the assessment of tolerance induction protocols in absence of a vascularized source of donor bone marrow cells. Further studies, however, are needed for achievement of stable tolerance induction of skin-containing allografts. The use of biologic agents, such as co-stimulatory blockade, may help to overcome this limitation and is discussed ahead in this chapter.
Nonhuman Primates
Tolerance induction regimens for solid-organ transplantation or VCA require testing in large animal models before clinical applicability, with NHPs representing an ideal preclinical model for the assessment of such protocols. The initial protocols tested in NHP included nonmyeloablative TBI, thymic irradiation, and T cell depletion. The severe T cell depletion obtained with these regimens, however, carried a high rate of mortality due to infections and development of lymphoma. In the attempt to reduce the toxicity of such regimens and obtain an effective T cell depletion, horse anti-thymocyte globulin (ATG), splenectomy, and a short course of cyclosporine A was added to these protocols, allowing for detection of high-level multilineage chimerism [44]. Unlike the long-term chimerism observed in mice studies, this protocol only allowed for transient chimerism. Surprisingly, despite cessation of cyclosporine and loss of mixed chimerism , the NHP did not reject its renal allograft, which was deemed viable for several years without any signs of rejection [44]. To reduce the toxicity of this protocol, the TBI was fractioned over 2 days. In this study, 11 of the 14 recipients developed transient mixed chimerism and eight of them survived long-term without need for any immunosuppressive drugs, with the longest kidney recipient surviving over 14 years with a functioning graft. Stable tolerance was confirmed using skin grafting, by evidence of acceptance of donor skin and prompt rejection of skin from third-party animals.
Similar protocols have been used in NHP to translate these encouraging findings from solid organ to reconstructive transplantation . NHP models offer relevant anatomical and immunological similarity to humans for preclinical studies. However, transplantation of full hand and face allografts is extremely challenging both surgically and from a postoperative management standpoint. Aiming to simulate a clinical VCA (e.g., a hand transplant) while reducing surgical level of complexity, costs, and discomfort to the animals, Cendales et al. have described a sensate osteomyocutaneous radial forearm flap. This flap was performed in 14 allogeneic transplants and entailed the use of an immunosuppressive regimen similar to those used in clinical transplantation, such as a calcineurin inhibitor (tacrolimus), an antiproliferative agent (mycophenolate mofetil), and methylprednisolone allowing for evaluation of VCA histology. Other VCA models in NHP were also investigated by Barth et al., who described a partial face transplant model by isolation of an osteomyocutaneous flap based on the common carotid artery and the internal and external jugular vein [5]. In this protocol, long-term survival of the grafts on tacrolimus monotherapy was associated with development of posttransplant lymphoproliferative disorder (PTLD). Later studies from the same group have shown that the addition of mycophenolate mofetil to the regimen prevented PTLD [6]. Furthermore, in this study, the authors investigated the role of vascularized bone marrow in this model, demonstrating that the lack of the vascularized bone segment was associated with acute rejection by 2 weeks [7]. The role of mixed chimerism or other cell-based approaches to induce tolerance in these models remains largely unknown.
Immunomodulatory Approaches
Alternative approaches to modulate the immune response have entailed the use of different biologic agents directed toward specific target molecules. Some of these drugs are intended to avoid acute rejection and, simultaneously, decrease sensitization to donor antigens, usually due to donor-specific antibody formation . The addition of these compounds to the conditioning regimens aimed to replace splenectomy as a way to improve graft survival. A high number of residual T cells remains in lymph nodes and in the spleen following the common conditioning regimens used in these studies, making sensitization likely. The addition of splenectomy in some protocols, such as the delayed kidney transplant model, has been shown to lead to long-term graft survival [45]. Replacing splenectomy with co-stimulatory blockade molecules such as anti-CD154 (also known as anti-CD40L) or anti-CD152 (also known as CTLA4) has been associated with high-level and long-lasting chimerism, leading to long-term graft survival [77].
The mechanism of action of co-stimulatory blockade molecules is linked to the modulation of T cell activation. In order for alloantigens to robustly activate naïve T cells, two signals are required. Following processing of donor antigen by the APCs in the context of the MHC, the first signal is delivered upon interaction of the TCR with the MHC/Ag complex. The second signal is a co-stimulatory signal that can be delivered by another surface molecule. Failure to produce co-stimulation via a second signal induces a state of T cell anergy [108] (Fig. 14.2a). The two co-stimulatory pathways best known to allow normal development and maintenance of immunity involve the CD80/CD86–CD28 and the CD40–CD40L pathway. In the CD80/CD86–CD28 second signal pathway, the CD80 (also known as B7.1) and CD86 (alternatively known as B7.2) molecules on the surface of APCs deliver a co-stimulatory signal by interacting with the CD28 cell surface proteins expressed on naïve T cells [24, 41] (Fig. 14.2b). Furthermore, following TCR interaction with the MHC/Ag complex present on the APC, CD40L expression is induced in T cells, allowing interaction with its receptor CD40, which results in upregulation of CD80 and CD86 by the APCs. Moreover, the CD80/CD86–CD28 interaction triggers upregulation of CD40L surface molecules when the TCR is occupied [24]. The redundancy of these mechanisms, and the fact that these two pathways regulate T-cell-dependent immune responses critically, hints at the importance of such interrelated pathways.
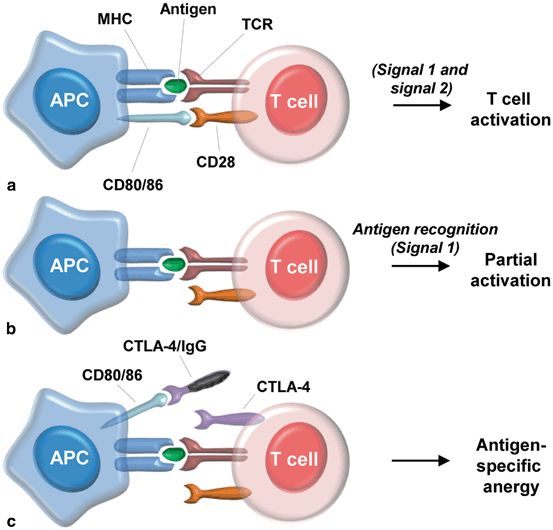
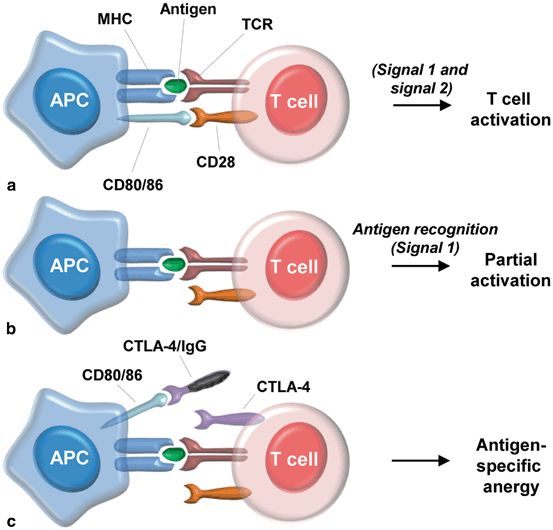
Fig. 14.2
T cell activation and co-stimulatory signals; a) T-cell activation via signal 1 and 2; b) Partial T cell activation by lack of signal 2; c) Antigen-specific anergy following co-stimulatory blockade. MHC: Major histocompatibility complex, CD: cluster of differentiation, APC: antigen-presenting cells, TCR: T cell antigen receptor; CTLA4-IgG: cytotoxic T-lymphocyte antigen 4-immunoglobulin G.
The presence of this co-stimulatory signal acts as a silencing signal, leading to T cell anergy and has been, therefore, used to promote tolerance induction. CTLA4 is similar to CD28 and therefore binds CD80/CD86, acting as a “switch off” signal. For this reason, much interest has developed around the use of biologic agents, such as monoclonal antibodies (mAb) targeting CD80/CD86 (named CTLA4-Ig) or targeting CD154, for tolerance induction in solid-organ transplantation. However, anti-CD40L mAb have displayed severe thrombogenic effects, limiting their clinical applicability [50
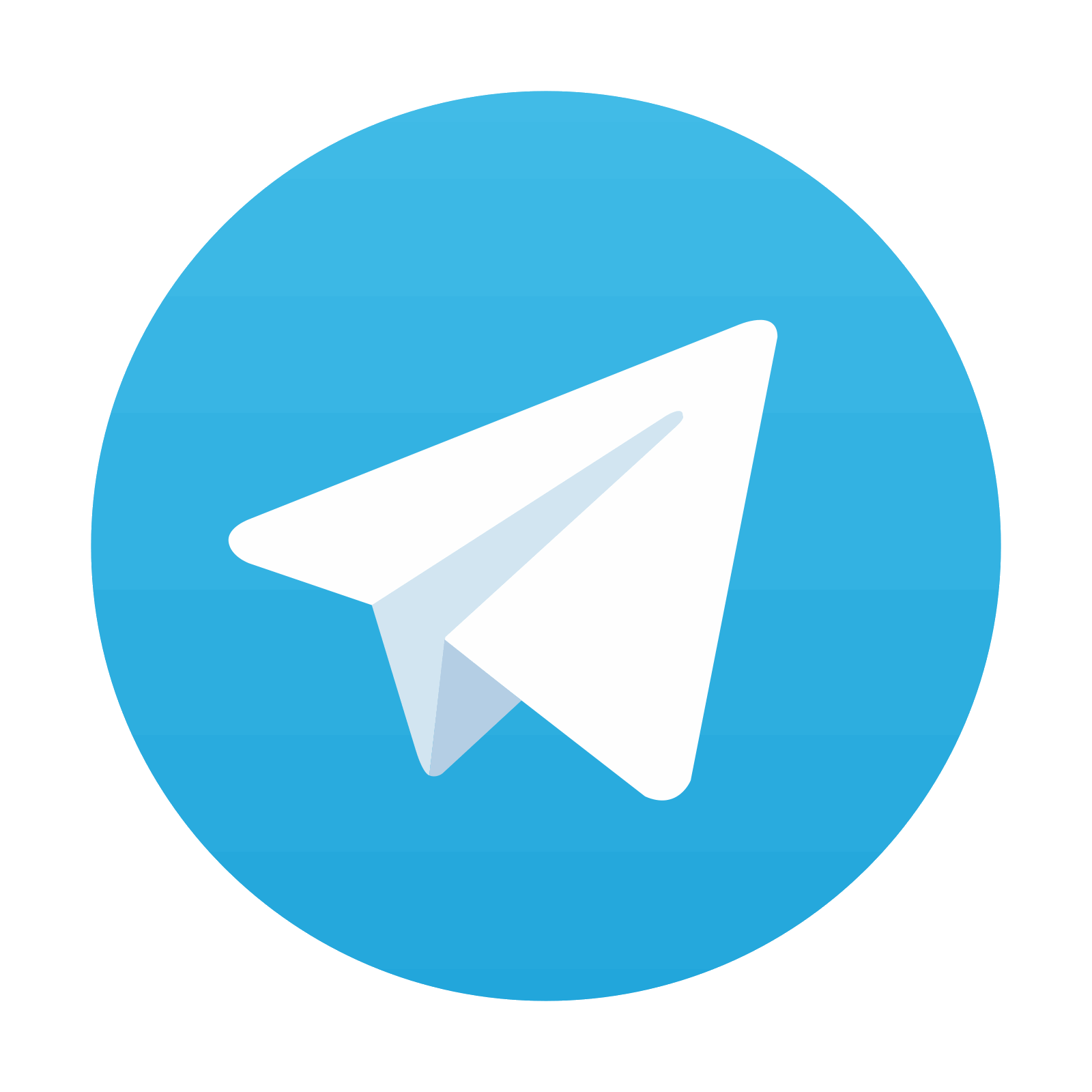
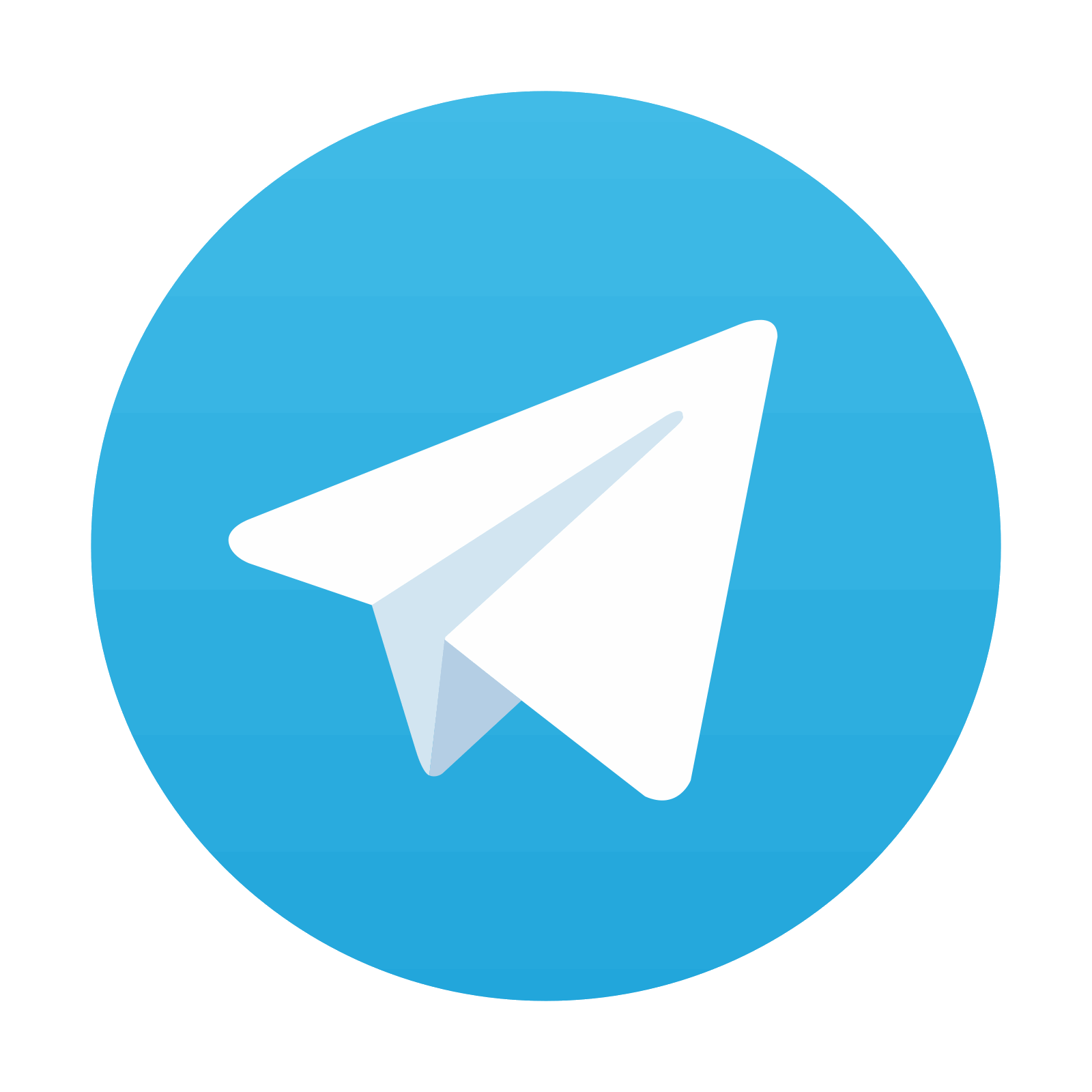
Stay updated, free articles. Join our Telegram channel
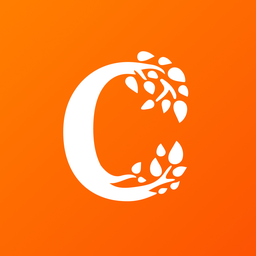
Full access? Get Clinical Tree
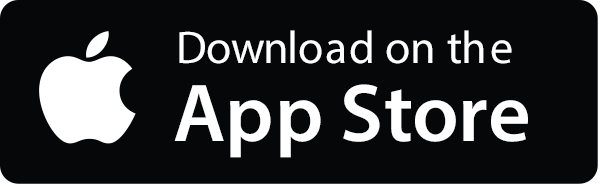
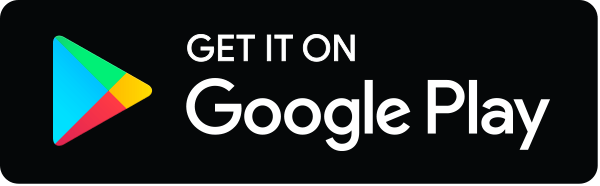