This article provides some insight into the basic science and concepts relevant to the use of local anesthetics by clinicians in the management of their patients, including a brief history of the development of local anesthetics and their physical properties, effectiveness, uses, limitations, and safety considerations. A generalized overview of the mechanism of action is also provided. The molecular detail of local anesthetics and voltage-gated ion channels can form the basis of understanding of (1) future developments in this area, and (2) toxicity. Most of the peer-reviewed literature related to this topic stems from work in adult humans and animals.
Key points
- •
Local anesthetics are efficacious for several plastic surgery procedures.
- •
Amino amides are the most commonly used local anesthetics.
- •
Potency, onset, and duration are related to lipid solubility and protein binding.
- •
Different agents have differing duration and onset.
- •
Local anesthetics block the voltage-gated sodium channel nonselectively.
- •
The voltage-gated sodium channel offers the potential for selectivity.
- •
Metabolism of amide local anesthetics depends on liver function.
- •
The addition of adjuvants can alter efficacy and safety.
- •
Local anesthetic systemic toxicity is life threatening.
- •
Bupivacaine in large doses is potentially fatal.
- •
Levobupivacaine and ropivacaine are safer agents and are suitable substitutes for bupivacaine.
- •
Where large doses of local anesthetic are used, lipid rescue kits, which can reduce morbidity and mortality, should be available.
Introduction
Local anesthetics are a major contributor to medical and dental practice throughout the world. No doubt most, if not all readers are aware of the effectiveness of this class of medication through personal experience. Provision and delivery of anesthesia and/or analgesia using local anesthetic can be accomplished in several ways. Local anesthetics have been administered as a field block, injection near minor or major nerves and plexuses, and injection into the epidural or intrathecal space. Other methods include transcutaneous application, tumescent anesthesia, and intravenous delivery.
Introduction
Local anesthetics are a major contributor to medical and dental practice throughout the world. No doubt most, if not all readers are aware of the effectiveness of this class of medication through personal experience. Provision and delivery of anesthesia and/or analgesia using local anesthetic can be accomplished in several ways. Local anesthetics have been administered as a field block, injection near minor or major nerves and plexuses, and injection into the epidural or intrathecal space. Other methods include transcutaneous application, tumescent anesthesia, and intravenous delivery.
History
Since the introduction of cocaine to medical practice in 1884, local anesthetics have been and continue to be a valuable tool in surgical practice. Since its isolation in 1855, cocaine was first synthesized in 1898. All synthetic local anesthetics are derivatives of cocaine. Many readers will recognize a handful of the 20 or so synthetic agents used historically. Like any pharmaceutical agent, there have been continued attempts to improve the efficacy and safety of this class of medication. Between 1891 and the 1970s several amino-ester (ester) and amino-amide (amide) local anesthetics have been synthesized. Two newer agents have since been developed and introduced, primarily to address improved safety.
Basic and clinical science of local anesthetics
Amides
The most versatile of the group continues to be lidocaine. Lidocaine and the majority of local anesthetics currently used in surgical practice are amides. First developed in 1943, it is functional, relatively rapid in onset and removed, safe, and its use is extensive. The vast majority of plastic surgeons have a working clinical knowledge of lidocaine use. Perhaps the second most commonly used agent is bupivacaine, first developed in 1957 and released for clinical use in 1963. It is more potent and longer acting than lidocaine, and provides suitable anesthesia and/or postprocedure pain control on the order of 2 to 4 times that of lidocaine. The major drawback to its use is safety. Several fatalities caused by local anesthetic systemic toxicity (LAST) have involved the use of bupivacaine.
Prilocaine was first synthesized in 1953. Its most common usage today is as a component of EMLA (a mixture of lidocaine and prilocaine). Some see this as a useful agent for intravenous regional anesthesia (IVRA). Prilocaine is also known for one of its toxic reactions, methemoglobinemia.
Mepivacaine, first synthesized in 1956, is similar to lidocaine with regard to onset and duration of action. It is thought to have some vasoconstrictive properties, which is a characteristic of few local anesthetics. Mepivacaine is a chiral molecule, and is sold as a racemic mixture of R and S optical isomers.
Articaine (synthesized in 1969) has extensive use in dental anesthesia. A PubMed search results in more than 300 references since the year 2000, most of which are related to dental practice, with some anesthesia studies. Its use in tumescent anesthesia for liposuction has been evaluated. Possible advantages are rapid onset and short duration, attributable to an additional ester group on the lipophilic side chain that is rapidly metabolized.
Esters
Cocaine is still used in medical practice, primarily in nasal surgery. Other agents with fewer side effects are available, but cocaine’s vasoconstrictive effects remain the primary reason for its use. Systemic absorption of cocaine can result in hemodynamic alteration in the patient.
Benzocaine, first synthesized in 1890, is not used in the clinical situation but is present in some over-the-counter cough drops.
Tetracaine was synthesized in the 1930s; its primary use is in topical anesthesia (ophthalmology) and in wound anesthesia.
Chlorprocaine, first introduced in 1955, is a rapid-onset, short-acting agent. Like most esters, it is rapidly metabolized by plasma esterases, so it is viewed as being very safe. In those with pseudocholinesterase deficiency, duration may be longer and the safety reduced. Since 2013, its availability in North America has been limited because of a manufacturing issue.
Newer Agents
For several years the agents listed thus far were the basis of local anesthesia. In response to fatalities involving bupivacaine, a search for a safer alternative was undertaken. Two commercially available amides were the result of this search: ropivacaine and levobupivacaine.
Synthesized in 1993 and introduced in 1996, ropivacaine is a single enantiomer. It has an improved risk profile in comparison with bupivacaine, and a similar duration of action. Ropivacaine does not cause the same degree of vasodilation as many local anesthetics. Use of ropivacaine in plastic surgery is limited, although its use has been evaluated in digital nerve blocks and infiltrative anesthesia.
Similarly, levobupivacaine is the S -enantiomer of bupivacaine. Released in the late 1990s, its safety profile is better than that of racemic bupivacaine. The clinical effect is similar to that of ropivacaine and bupivacaine, but studies do exist demonstrating superiority of one over the other. Much of the research regarding comparison with ropivacaine has been in peripheral nerve block and neuraxial anesthesia/analgesia.
The difference between agents is frequently identified as potency. Lack of familiarity, cost, and limited improvement on currently available local anesthetics have hindered the adoption of these 2 agents. However, in situations where large doses of local anesthetic are used, either of these newer agents should be considered.
Mixing of Local Anesthetics
Depending on the intended use of a local anesthetic, a clinician may look at the various properties of a particular anesthetic (speed of onset, duration, dosage, toxicity, and so forth) and “wish” for an improved picture. The mixing of local anesthetics has been evaluated, with varying results. Caution should be exercised when mixing agents because the risk of error is possible. The practice of mixing agents is most commonly used in peripheral nerve block.
Structure
Synthetic local anesthetics are all derivatives of cocaine, are small molecules with molecular weights of less than 500, and fall primarily into 2 categories ( Fig. 1 ). A lipophilic portion and a hydrophilic portion are linked by either an amino ester or an amide. Modification to either of the side chains can change the physical properties of the agent. For agents with a chiral carbon (ie, mepivacaine and bupivacaine) it is possible to separate racemic mixtures to realize a single optical isomer. The isolated optical isomer is one of the more recent advances to show potential for clinical use.
Mechanism of Action
Many readers will be familiar with the concepts of transmembrane potential and the action potential in excitatory cells. This action potential is the unit of information transfer in the motor, sensory, or autonomic nervous system. Sodium flux across the cellular membrane is a primary contributor to the development and propagation of the action potential. Local anesthetics reduce or stop sodium movement across the membrane by blocking the voltage-gated sodium channel. This blockade of the sodium channel is accepted as the mechanism whereby local anesthetics work. When enough sodium channels are blocked, neural traffic is stopped and anesthesia results. These agents also cause nonselective blockade of potassium and/or calcium channels. These secondary effects contribute to side effects and toxicity.
The Sodium Channel
To a clinical physician, the significance of this molecular-complex voltage-gated sodium may not be readily apparent. However, the structure and function of these channels is a basis for understanding the mechanisms, complications, and future developments of local anesthetics. The sodium channel is composed of 1 α and 2 β subunits. The α subunit is further divided into 4 domains, and each domain has 6 transmembrane segments ( Fig. 2 ). Often represented as a 2-dimensional linear structure across the cell membrane, in vivo its 3-dimensional structure forms a channel. Two of the transmembranal segments (S5 and S6) from each domain contribute to a channel which, when open, allows the sodium ion to traverse the membrane. The regulation of this channel’s patency is determined by a voltage-sensitive (charged) portion of a segment from each domain. In a changing electrical field, such as occurs during the action potential, a conformational change in the channel results in closing or opening of that channel. Local anesthetics bind to a segment of the α subunit (segment 6 of domain 4). When this occurs the channel does not open, regardless of voltage change.
The voltage-gated sodium channel exists in 3 conformational states. The “neutral” state can be considered the resting closed state. Transient changes in transmembrane voltage (toward less negative) results in a conformational change to the activated open state. During this time there is significant ion movement and subsequent regional alteration in the transmembrane potential, which results in nearby voltage-gated channels moving to the activated open state and propagation of the action potential. As the transmembrane potential returns to its baseline state through the movement of primarily potassium ions, the channel changes to an inactivated closed state, by way of a voltage-sensitive mechanism.
Local anesthetics bind to a receptor on the sixth segment of the fourth domain, which causes the channel to become stabilized in the inactivated closed state. Movement of sodium ions is thus halted, regardless of changes in transmembrane potential. The result is failure of the action potential to propagate, and potential sensory and motor information is not transmitted.
For an agent to access the receptor site it must enter the cell. Larger molecules, such as pufferfish toxin, block sodium conduction from the outside of the cell. There is specificity for various sodium channels, and the blockade is probably a physical obstruction rather than the conformational change that results from the action of local anesthetic. To enter the cell requires movement across the bilipid cell membrane. Physical properties, in particular the lipophilicity of a particular local anesthetic, will determine the amount and speed at which this movement occurs.
Potency of Local Anesthetics
In general terms, local anesthetics have a low affinity for the sodium channel. The relatively large doses account for the effectiveness of the agents. Potency describes the relative activity of 2 medications with the same mechanism of action. A medication is more potent if a lesser “amount” of an agent is required to produce the same effect when compared with the other. Considering the 2 most commonly used agents, lidocaine and bupivacaine, the latter is more potent. A major contributor is lipid solubility ( Table 1 ). A related phenomenon is the duration of action. Generally speaking, more potent agents have a longer duration of action ( Table 2 ). With respect to bupivacaine, its greater toxicity is related to potency.
Local Anesthetic | Toxicity | Lipid Solubility | Protein Binding (%) | Potency | p K a | Onset | Duration of Action | |
---|---|---|---|---|---|---|---|---|
Relative | Hours | |||||||
Cocaine | High | — | 98 | Low | 8.7 | Slow | Long | — |
2-Chlorprocaine | Low | Low | 0 | High | 9.1 | Very rapid | Short | 0.5–1 |
Tetracaine | High | High | 76 | High | 8.4 | Slow | Long | 2–6 |
Articaine | Low | High | 94 | — | 7.8 | Rapid | Short | 1 |
Bupivacaine | High | High | 96 | High | 8.1 | Slow | Long | 3–8 |
Lidocaine | Low | Low | 65 | Low | 7.8 | Rapid | Medium | 1–3 |
Levobupivacaine | Intermediate | High | 96 | High | 8.1 | Moderate | Long | 3–8 |
Mepivacaine | Intermediate | Low | 78 | Low | 7.7 | Moderate | Medium | 2–4 |
Prilocaine | Intermediate | Low | 55 | Low | 8.0 | — | Medium | — |
Ropivacaine | Intermediate | Intermediate | 93 | High | 8.1 | Moderate | Long | 3–8 |
Local Anesthetic Preparations Concentration in Volume % (mass/mL) | Maximal Single Dosing by Mass or Volume | Expected Duration by Site or Administration Appropriate Dose (hours) | ||||
---|---|---|---|---|---|---|
mg/kg | Maximal Dose (mg) | Max Volume (mL) | Infiltration (min) | PNB (min) | Epidural (min) | |
Lidocaine Plain | ||||||
0.5% (5mg/mL) | 5 | 200–300 | 40–60 | 75–90 | 90–120 | 30–45 |
1% (10 mg/mL) | 5 | 200–300 | 20–30 | 75–90 | 90–120 | 30–45 |
Lidocaine plus epinephrine | there is 5 mcg/mL of epinephrine in commercial preparation of lidocaine +epi | |||||
0.5% (5 mg/mL) + epinephrine | 7 | 500 | 100 | 90–180 | 120–240 | 80–120 |
1% (10 mg/mL) + epinephrine | 7 | 500 | 50 | 90–180 | 120–240 | 120–180 |
Buupivacaine | there is 5 mcg/mL of epinephrine in commercial preparation of bupivacaine + epi | |||||
0.25% (2.5 mg/mL) | 2.0 | 175 | 70 | 180–360 | 360–720 | 165–225 |
0.5% (5 mg/mL) | 2.0 | 175 | 35 | 180–360 | 360–720 | 165–225 |
0.25% (2.5 mg/mL) + epi | 3.0 | 225 | 90 | 200–400 | 360–720 | 165–225 |
Ropivacaine/Levobupivacaine | the addition of epinephrine does not affect maximal dose or duration | |||||
0.5% (5mg/mL) | 3.0 | 225–300 | 45–60 | 180–240 | 360–720 | 165–225 |
ropivacaine 0.5% + epi | 3.0 | 225–300 | 45–60 | 180–240 | 360–720 | 165–225 |
levobupivacaine 0.5% | 3.0 | 225–300 | 45–60 | NA | 360–720 | 165–225 |
Tumescent lidocaine with epinephrine | Preparation involves diluting of plain lidocaine with with normal saline and the addition of epinephrine (0.5–1 mcg/mL) and sodium bicabonate to 1000 mLs | |||||
0.05–.1% (0.5–1 mg/mL) | 55 | 3500 a | 3500–7000 | 480–1200 | — | — |
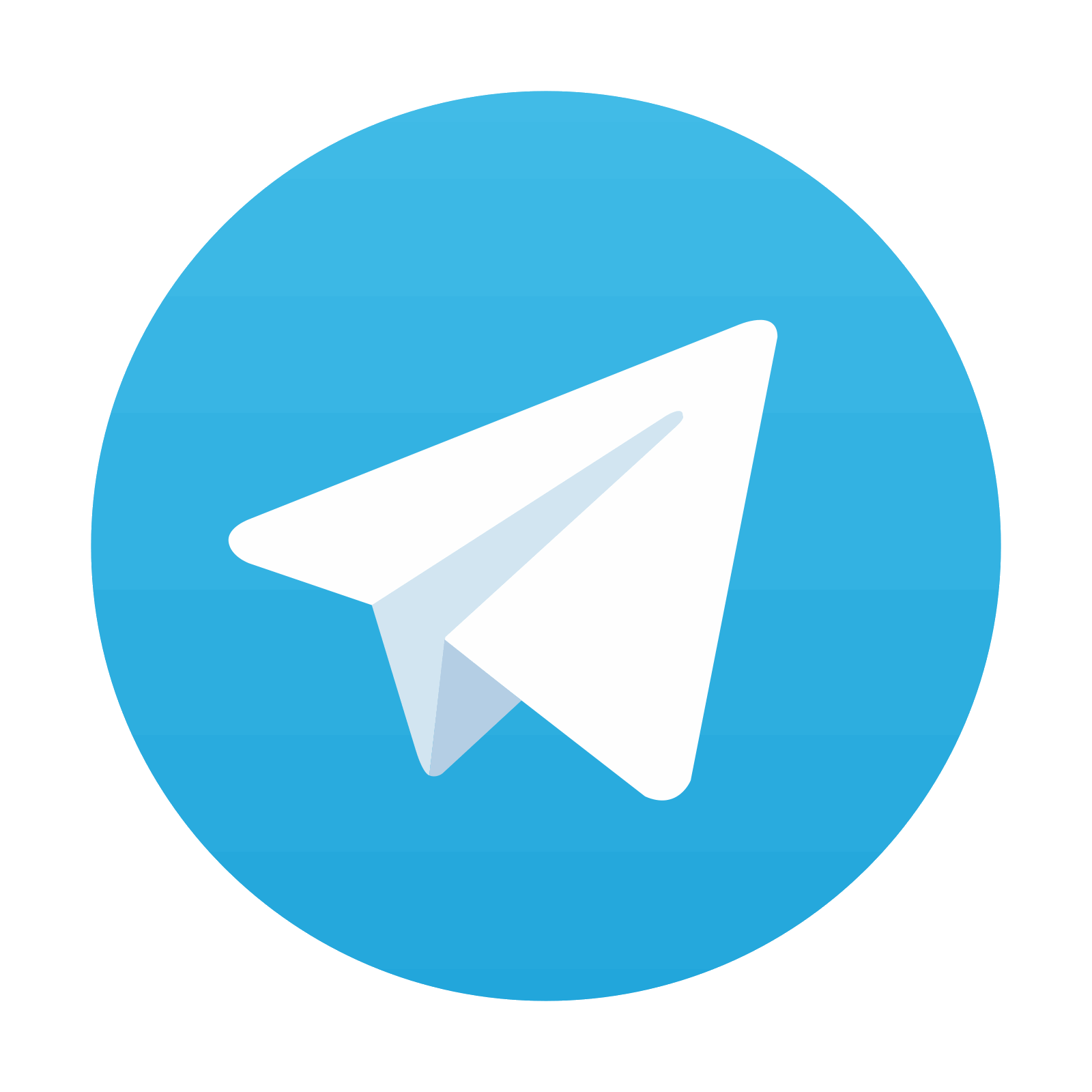
Stay updated, free articles. Join our Telegram channel
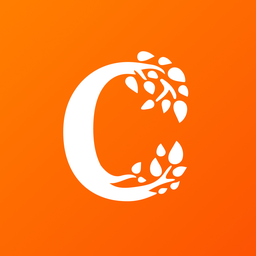
Full access? Get Clinical Tree
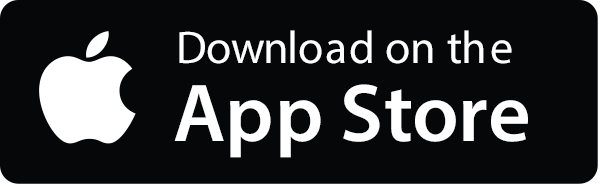
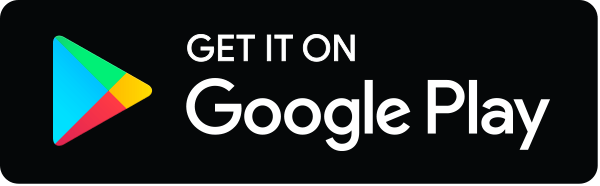
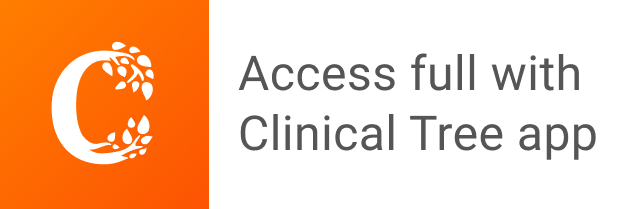