Fig. 2.1
Epidermal structures. Insert, electron micrograph: Murine skin was fixed in Karnovsky’s fixative overnight, and postfixed with 0.25 % ruthenium tetroxide. Ultrathin sections were examined using an electron microscope
2.2 Stratum Corneum Structure
The stratum corneum is composed of two compartments, corneocytes and lipid-dominant extracellular lamellar membrane structures (Fig. 2.1). The architecture of the stratum corneum is referred to as “brick (corneocyte) and mortar (lamellar membranes).” Approximately 15 layers (20 μm) of corneocytes, denucleated forms of keratinocytes, are present in the normal human stratum corneum (with ≈ 200 layers on the sole and palm). In corneocytes, the plasma membranes are replaced by protein cross-linked cornified envelopes, which differs from nucleated cells surrounded by plasma membranes that comprise a lipid bilayer. Although de novo syntheses of proteins, lipids, and nucleotides do not occur in the stratum corneum, these existing cellular components and likely exogenous compounds are catabolized in the stratum corneum.
2.2.1 Corneocyte
During the transition from granular layers to stratum corneum, nuclei of keratinocytes are degraded. Denucleated keratinocytes, i.e., corneocytes, exhibit a flat shape and are filled with keratin fibers and degraded products of proteins, lipids, and nuclei. Endogenous humectant’s natural moisturizing factor (NMF) is generated in the stratum corneum by degradation of histidine-rich proteins, primarily filaggrin [2]. Filaggrin deficiencies are due to mutations of the filaggrin gene associated with ichthyosis vulgaris and atopic dermatitis [3]. The filaggrin deficiencies cause decreased NMF and therefore declining hydration in the stratum corneum [4].
Corneodesmosomes comprised of desmoglein-1 and desmocollin-1 attach to other corneocytes [5]. Corneodesmosomes are degraded by an acidic pH optimum aspartyl protease, cathepsin D, in the stratum corneum [6] and neutral pH optimum chymotriptic- and tryptic-serine proteases [7–9], including kallikrein-related peptidases, which results in shedding corneocytes from the epidermis, i.e., desquamation. The kazal-type family (SPINK) is endogenous trypsin-like and chymotrypsin-like serine protease inhibitors that are present in the stratum corneum and are involved in the regulation of desquamation [10–12]. Netherton syndrome, a severe autosomal recessive ichthyosis, showing abnormal desquamation, is caused by a loss-of-function mutation in SPINK5, which encodes the lymphoepithelial kazal-type trypsin inhibitor (LEKT1) [13].
2.2.2 Cornified Envelope
Nucleated cells are surrounded by a plasma membrane consisting of lipid bilayer structures, and plasma membranes are replaced by a cornified envelope that is formed by cross-linked proteins [14]. The synthesis of constituted proteins of the cornified envelope, i.e., loricrin, involucrin, small proline-rich proteins (SPRs), envoplakin, and other minor protein components, increase at late stages of epidermal differentiation. Protein cross-linkages are due mainly to the ε-(γ-glutamyl) lysine isopeptide bond generated by transglutaminases (TG) [15]. Seven isoforms of transglutaminases are characterized in mammals. Five isoforms, TG1, TG2, TG3, TG5, and TG6 are expressed in keratinocytes [15–17]. In particular, TG1, which is a major isoform in keratinocytes [15], is critical for cornified envelope formation [18]. The cornified envelope exhibits a stable rigid property to resist mechanical barrier stress [19]. Mutations of the TG1 gene have been shown in lamellar ichthyosis [20], bathing suit ichthyosis [21], and congenital ichthyosiform erythroderma [22], and a TGM5 mutation is associated with peeling skin syndrome [23].
2.2.3 Corneocyte Lipid Envelope
The outer leaflet of the cornified envelope is covered by a monolayer of corneocyte lipid envelope (CLE), which consists of omega (ω)-hydroxy-ceramides and its catabolites, ω-hydroxy free fatty acid generated by ceramidase [24–26] (Fig. 2.2). CLE is formed as follows: (1) ω-O-acyl residue is released from ω-O-acyl (predominantly an essential fatty acid, linoleate) glucosylceramides; (2) ω-hydroxyl residue of ω-hydroxy-glucosylceramides is covalently bound to cornified envelope proteins (primarily to glutamate residues in cornified envelope protein [mainly involucrin]) [27]; (3) ω-hydroxy-glucosylceramides are deglucosylated by ß-glucocerbrosidase to cornified envelope-ω-hydroxy-ceramides; (4) some CE-ω-hydroxy-ceramides are hydrolyzed to cornified envelope-ω-hydroxy free fatty acid by ceramidase(s) [26, 28]. Releasing of linolate residue of ω-O-linoleoyl glucosylceramides is required by 12R lipoxygenase (12R-LOX) or epidermal lipoxygenase 3 enzymes, which oxidize the linoleate moiety of ω-O-linoleoyl glucosylceramides, generating an oxidized species that subsequently attach to cornified envelope proteins [29]. Mutation of these lipoxygenases is associated with nonbullous congenital ichthyosiform erythroderma [30]. TG1 is involved in lipid and protein binding. Yet, CLE is evident in the stratum corneum of lamellar ichthyosis patients that show trace levels of TG1 activities [25]. Hence, other transglutaminase(s), other enzyme(s), or nonenzymatic transterification might also serve in CLE formation [25]. A role for CLE has been proposed as a scaffold to form lamellar membrane structures (see below, Sect. 2.4) [24, 25]. It is also possible that CLE regulates egress of hydrophilic substances from corneocytes. Yet, the roles of CLE in the stratum corneum are still largely unknown.
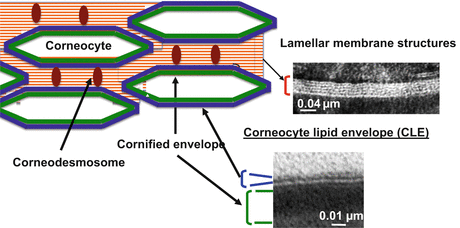
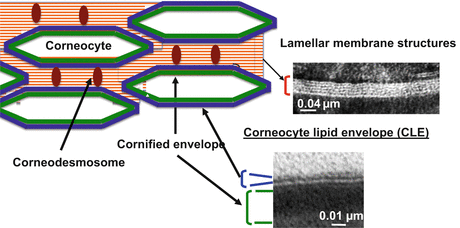
Fig. 2.2
Stratum corneum structure. Cornified envelope, Green; Corneocyte lipid envelope (CLE), Blue; Corneodesmosom, Brown. Insert, electron micrograph: Murine skin was fixed in Karnovsky’s fixative, and post-fixed with 1 % aqueous osmium tetroxide, containing 1.5 % potassium ferrocyanide
2.2.4 Lamellar Membrane Structure
In contrast to dermis in which extracellular matrix is filled with collagen, elastin, glycosaminoglycans, and glycoproteins, lipid-enriched lamellar membrane structures fill the extracellular domain in the stratum corneum. Lamellar membranes extend in a horizontal direction (Figs. 2.1 and 2.2). Corneodesmosomes disengage from the lamellar membrane structure to bind to other corneocytes. Corneodesmosomes increase integrity and also regulate desquamation by their degradation.
Ceramide, cholesterol, and free fatty acid are major constituents (95>% of total lipid) [31] of lamellar membrane structures. Ceramide metabolites, sphingosine [31], and ceramide-1-phosphate [32] are present as minor components. Ceramides in the stratum corneum comprise at least 12 molecular groups of heterogeneous molecular species, including epidermal unique ultralong chain (up to 34 carbon chain lengths) fatty acid, ultralong chain omega-hydroxylated fatty acid, and ultralong chain omega-O-acylated molecular species [33, 34]. These ceramides are produced from their immediate precursors, glucosylceramides and sphingomyelins, by ß-glucoceramidase and sphingomyelinase, respectively, at the transition from granular layer to stratum corneum [35, 36] (Fig. 2.1). Most of these precursor lipids are sequestrated in the epidermal lamellar body in the stratum granulosum [37, 38]. Incorporation of glucosylaceramide into lamellar bodies requires ABCA12 (ATP binding cassette transporter, family 12). A devastating ichthyosis, Harlequin ichthyosis, due to ABCA12 mutations, leads to abnormalities in lamellar body formation, lamellar membrane structures in the stratum corneum, and in permeability barrier function [39].
Similar to ceramide, most free fatty acids are generated from their immediate precursor lipids, i.e., glycerophospholipid and triacylglycerol, by phospholipase(s) and triglyceride lipase, respectively. In addition, a pool of cholesterol is also produced from cholesterol sulfate and cholesterol esters by cholesterol sulfatase and cholesterol esterase, respectively. Cholesterol sulfatase deficiency is the pathogenesis for X-linked ichthyosis [40].
Electron microscopic analysis characterizes lamellar membrane structures, whereas X-ray diffraction, neutron diffraction, FT-IR (Fourier transform infrared spectroscopy), and DSC (Differential scanning calorimetry) analyses reveal further details of lamellar membrane structures in the stratum corneum. Two lamellar phases with periodicities of approximately 6 nm (short-periodicity phase) and 13 nm (long-periodicity phase) are present [41]. Intracorneocyte soft keratin is a major reservoir of water in the stratum corneum [42]. In addition, a small angle neutron scattering analysis demonstrated the swallowing of the lipid lamellar structures following increased humidity, indicating the presence of water in lamellar membrane structures [43], and a recent small-angle diffraction study elucidated that the short-periodicity phase is a slightly altered periodicity phase following incorporation of water [42]. In addition to two-dimensional structures of lamellar membrane structures, three-dimensional structures representing lamellar packing (hydrocarbon packing) have been characterized. Both hexagonal and tightly packed orthorhombic structures are present in the stratum corneum. Moreover, a recent study using low flux electron diffraction analysis indicated the presence of a different type of orthorhombic structure showing a different distance of packing space, in the lamellar membrane structures in the stratum corneum [44]. The alteration of the lamellar organization has been shown in skin diseases associated with compromised permeability barrier function (e.g. atopic dermatitis) [45].
2.3 pH in the Stratum Corneum
The pH of nucleated cellular layers is neutral, whereas the stratum corneum is acidified.
The acidification is due to four major groups of acidic components derived from keratinocytes. First, free fatty acid generated from both de novo synthesis and hydrolysis of esterified lipids such as triacylglycerol, glycerophospholipids, and cholesterol esters. Second, cholesterol sulfate and sulfate following hydrolysis by cholesterol sulfatase contribute to acidification [46]. Ceramides are hydrolyzed to free fatty acid and sphingoid base by ceramidases, which are present in the stratum corneum [47–49]. However, because sphingoid bases are alkaline, ceramide hydrolysis is unlikely to contribute to acidification. Third, urocanic acid is produced from histidine (mainly from NMF) by histidase (See below, Sect. 2.5.2). And finally, proton (H+) is discharged from keratinocytes through Na+/H+ antiporter [50]. In addition to keratinocyte-derived components, sweat-derived lactate, metabolites of microorganisms, and chemicals from air also contribute acidification to the stratum corneum. Acidification contributes to form the antimicrobial barrier and also regulates enzyme activity in the stratum corneum.
Most prior studies measured skin surface pH or a different layer following tape stripping using a flat pH electrode, although recent technologies, confocal fluorescence microscopy, and fluorescence life-time imaging (FLIM) with a development of pH sensitive florescence dye allow us to observe major pH distribution in the stratum corneum, and also in intracellular and extracellular domains [51]. The pH gradient appears to be present across the stratum corneum; i.e., decreasing pH toward skin surface [51, 52], and recent studies show an opposite trend of pH gradient [53]. Hence, the presence of pH gradient and whether continuous acidification occurs in the stratum corneum still remains to be resolved.
2.4 Barrier Function
Stratum corneum deploys multiple barrier functions to protect internal cells and tissues from external perturbations while maintaining the internal environment and normal cellular functions (Table 2.1).
Table 2.1
Barrier roles of stratum corneum in maintaining epidermal homeostasis
Barrier | Roles | Effectors |
---|---|---|
Permeability | Prevents excess water loss | Primarily extracellular lamellar membrane structures |
Maintains body temperature | ||
Prevents ingress of xenotoxic chemicals and allergens | ||
Prevents invasion of microbes | ||
Antimicrobial | Protects against diverse microbes (gram-positive and gram-negative bacteria, fungi, and viruses) | Antimicrobial peptides |
Acidic pH | ||
Sphingoid bases | ||
Antioxidant | Protects epidermis from oxidative stress | α-/γ-tocophenol |
Ascorbic acid | ||
Glutathione | ||
Mechanical | Protects epidermis from mechanical stress | Primarily cornified envelopes |
UV | Protects epidermis from cell death, DNA damage, and oxidative stress | Urocanic acid |
Structural components (proteins, lipid, nucleotides) |
2.4.1 Epidermal Permeability Barrier
The epidermal permeability barrier prevents both egress and ingress of substances. Blocking excess water evaporation from nucleated layers of epidermis is critical for not only dehydration, but also maintaining body temperatures. Prevention of ingress of xenotoxic chemicals, allergens, and microbial pathogens is also an essential function of the epidermal permeability barrier. Substances of larger than 500 kDa are unable to penetrate into the stratum corneum [54]. Recent studies using liposome and nanoparticles demonstrate the penetration of larger sizes of molecules into the nucleated layer of cells. Penetration of nanoparticles into the stratum corneum is not completely understood.
2.4.2 Antimicrobial Barrier
Acidification of the stratum corneum increases antimicrobial defense. For instance, with pH below 5.5, the growth of Pseudomonas acne, Staphyrococcus epidermidis, and a virulent microbial pathogen, Staphylococcus aureus, are suppressed [55]. In addition, sphingoid bases generated from ceramide by ceramidases show antimicrobial activities in vitro [56–58]. Moreover, an innate immune component, antimicrobial peptides, is present in the stratum corneum to combat broad ranges of microbes (see Sect. 2.5).
2.4.3 Antioxidant Barrier
2.4.4 UV Barrier
Longer wavelengths of UV, i.e., UVA (315–340 nm), reach to the dermis, whereas most lower UV wavelengths, i.e., UVB (280–315 nm), are absorbed in the epidermis. Urocanic acid, which is generated from histidine, has been shown to be an epidermal major chromophor, i.e., a potent endogenous UV absorbent (See Table 2.1) [60]. In addition, because the bulk amounts of proteins, lipids, and nucleotides, which are not potent chromophors, are abundant in the stratum corneum, these components could contribute to forming the UV barrier.
2.5 Roles of Stratum Corneum in Immunity
2.5.1 Innate Immunity
Antimicrobial peptides, small, cationic (some of them are anionic, e.g., dermcidin), amphipathic molecules, are a part of the host innate immunity forming the antimicrobial barrier [61, 62]. Antimicrobial peptides display potent antimicrobial activities against a broad range of microbes, including gram-negative and gram-positive bacteria, fungi, and some viruses [63, 64]. Antimicrobial peptides are synthesized in nucleated layers of keratinocytes, infiltrate immune cells in the skin, and are retained in the stratum corneum.
2.5.1.1 Cathelicidin
Cathelicidin antimicrobial peptide (CAMP) is synthesized as the inactive precursor protein CAP18, followed by proteolytic digestion yielding an active antimicrobial peptide, 37-amino-acid peptide (LL-37), i.e., 37 amino sequences of C-terminal of CAMP [64]. CAMP/LL-37 is inducible with infection, injury or inflammatory response [64–66]. CAMP expression is regulated by 1,25 dihydroxy vitamin D3-mediated vitamin D receptor (VDR) activation [65, 66]. In addition, subtoxic external perturbations such as UV-B irradiation and acute barrier disruption trigger endoplasmic reticulum (ER) stress to stimulate the production of a signal lipid, sphingosine-1-phosphate (S1P) that induces CAMP production via NF-κB-C/EBPα activation, independent of the 1,25 dihydroxy vitamin D3-mediated mechanism [67]. Note that S1P→NF-κB-dependent mechanisms are primarily operated under stressed conditions, which suppress VDR-dependent transcriptional activity [68]. Hence, both S1P→NF-κB- and VDR-dependent pathways could complementarily regulate CAMP expression to maintain antimicrobial defense.
CAMP is a multifunctional AMP. CAMP modulates epidermal immune function, i.e., stimulating cytokine production/secretion including inflammatory and cellular migration [69–72]. Excess CAMP/LL-37 expression as well as hydrolytic peptides of LL-37 are involved in inflammatory responses in rosacea [73, 74].
2.5.1.2 Defensins
The defensins are categorized in three subfamilies, α-, β-, and θ-defensin [75, 76]. Four human β-defensins (hBD1, hBD2, hBD3, and hBD4) are expressed in KC, and β- and θ-defensins are mainly produced by neutrophils and bone marrow, respectively. hBD1 is constitutively expressed in epithelial cells, including KC, whereas hBD2, hBD3, and hBD4 are inducible peptides in epidermis in response to microbial infection, inflammation, and differentiation [75, 77, 78]. hBD2 expression is increased in inflamed skin and is induced by IL-1α and IL-1β, whereas hBD3 is induced by IL-6 and epidermal growth factors. hBD4 expression is stimulated in response to phobol 12-myristate 13-acetate (PMA) or calcium [75, 78]. Activation of toll-like receptor (TLR) 4 also induces expression of hBD2, but not hBD3, in KC [79].
2.5.2 Adaptive Immunity
The trans form of urocanic acid is produced from histidine (mainly from NMF) by histidase. The trans form converts to cis form by UV irradiation. Because urocanic acid is a potent chromophor, topical urocanic acid was used as a natural-occurring, apparently safe UV absorbent in skin care products. However, immunosuppression effects of cis urocanic acid were found [83], and topical cis urocanic acid was found to increase skin cancer risk in murine skin [84]. Thus, urocanic acid is no longer formulated in skin care products. Cis urocanic acid binds to the serotonin [5-hydroxytryptamine (5-HT)] receptor to suppress immune function [85]. Moreover, recent studies show that cis urocanic acid activates T-regulatory cells [86, 87]. However, it is unclear if urocanic acid generated in the stratum corneum is transferred to the nucleated cellular layer of epidermis to suppress immunity.
Increased DNA damage following UV irradiation was evident in histidase-deficient mice compared with wild-type mice [88], and it has been proposed that trans-urocanic acid decreases DNA damage by thymidine dimer formation. However, it is unclear what the different roles of both trans- and cis-urocanic acid in skin are.
2.6 Conclusion and Perspective
The stratum corneum is located at the interface of the external and internal environment, two environments that have different features, e.g., humidity, temperature, and osmolarity. The stratum corneum deploys protective barrier mechanisms to minimize the impact of the external environment on internal cell/tissue, so their normal functions can be maintained. Most stratum corneum structures and their constituents are unique in forming this competent barrier. Necessary redundancies of constituents in the stratum corneum contribute to the maintenance of cutaneous and extracutaneous homeostasis. Most cellular components in the stratum corneum are well-designed and their syntheses are well-regulated. The denucleation of keratinocytes to become corneocytes should be a strategy to prevent DNA damage, inasmuch as the repairing of DNA damage attenuates efforts devoted to high-priority tasks, i.e., the barrier function, in the stratum corneum. Utilization of saturated fatty acyl species to synthesize ceramide in the stratum corneum should also be a strategy to increase the stability of lamellar membrane structure against oxidative stress. The stratum corneum likely has another function: to act as a sensor of the external environment. This function needs to be further characterized. Compromised barriers influence living layers of epidermis leading to pathogenic effects, such as cell death and inflammatory responses. Inflammation alters normal keratinocyte proliferation and differentiation, resulting in attenuation of barrier formation to further decrease barrier functions. This spiral leads to chronic inflammation, delayed wound healing, infections, xerosis, and accelerated skin aging. Intervention in this spiral provides a therapeutic approach to these conditions. A barrier repair approach has been used for treatment of atopic dermatitis, in combination with anti-inflammatory medication. Characterization of the structures and their constituents in the stratum corneum, as well as their regulatory system, is a basis for developing therapeutic approaches. The importance of three stratum corneum lipids (ceramide, cholesterol, and free fatty acid) has been widely acknowledged. Yet, a recent study illuminates a minor ceramide catabolite, sphingoid base, which also contributes to and influences lamellar membrane structures in the stratum corneum, suggesting that further studies of previously uncharacterized/undefined structures, constituents, and their metabolism and roles in the stratum corneum will allow us to develop more potent therapeutic approaches for cutaneous diseases.
Acknowledgments
The author gratefully acknowledges Dr. Anna Celli, Dr. Peter M. Elias, and Dr. Theodora Mauro (Department of Dermatology, University of California, San Francisco and Department of Veterans Affairs Medical Center San Francisco, CA), Dr. Ichiro Hatta (Japan Synchrotron Radiation Research Institute/SPring-8), and Dr. Mitsuhiro Denda (Shiseido Research Center, Yokohama, Japan) for numerous critical discussions. The author acknowledges the superb editorial assistance of Ms. Joan Wakefield (Department of Veterans Affairs Medical Center San Francisco, CA). This study was supported by National Institute of Health grants AR051077 and AR062025 (the National Institute of Arthritis and Musculoskeletal and Skin Diseases) and a National Rosacea Society Grant.
References
1.
2.
Scott IR, Harding CR, Barrett JG (1982) Histidine-rich protein of the keratohyalin granules. Source of the free amino acids, urocanic acid and pyrrolidone carboxylic acid in the stratum corneum. Biochim Biophys Acta 719:110–117CrossRefPubMed
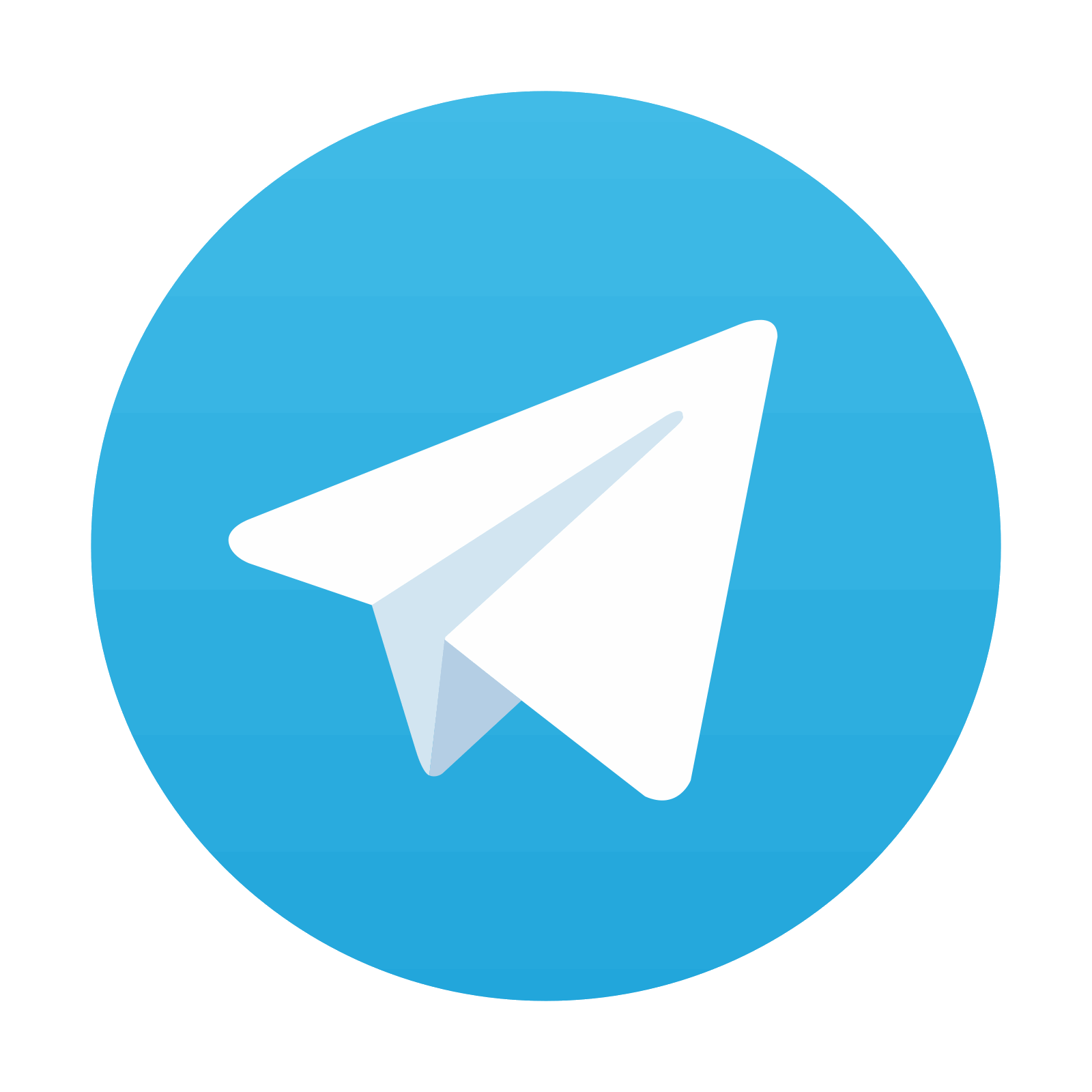
Stay updated, free articles. Join our Telegram channel
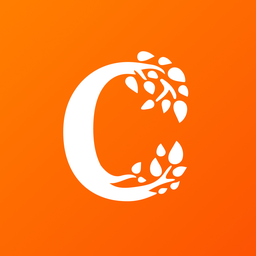
Full access? Get Clinical Tree
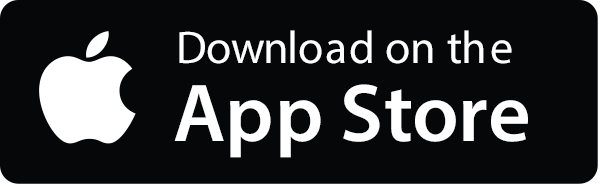
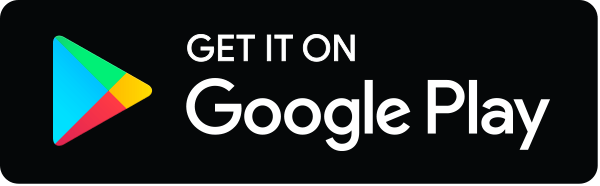