Fig. 12.1
Linear (Ia) and folded (Ib) structures of squalene (I) and structure of 2,3-oxidosqualene (II, squalene epoxide). Squalene is transformed into several oxygenated derivatives and breakdown compounds by different stressors (Ultra violet (UV), environmental oxidants, skin microflora). Enzymatic oxygenation of squalene to 2,3-oxidosqualene is catalyzed by squalene monooxygenase enzyme, consuming a mole of NADPH and one of oxygen
The lipogenic activity of the SG is finely regulated and depends partly on lipid signaling. Similarly to other lipogenic tissues the SG expresses liver X receptors (LXRs), which are members of nuclear receptor superfamily (Hong et al. 2008; Russell et al. 2007). LXRs staining has been detected within the nucleus of sebocytes. LXRs are key players of the lipid homeostasis acting as sensors of cholesterol levels. Upon binding to oxysterols, LXRs form dimers with the retinoid X receptor alpha (RXRα) and binds consensus sequences within promoters of target genes that regulate cholesterol and phospholipids efflux (Schultz et al. 2000). The expression of sterol regulatory element binding protein 1 (SREBP-1) is under direct control of LXRs following heterodimerization with RXRα (Swinnen et al. 1997). Detection of LXRα and LXRβ receptors in cultured sebocytes has led to investigate LXR agonists for their effects on cell proliferation and sebogenesis (Russell et al. 2007). Sterol regulatory element binding proteins (SREBPs) affect the expression of genes for both fatty acid and cholesterol synthesis. In particular, SREBP-1 is increased in SEB-1 human sebocyte in culture following stimulation with insulin-like growth factor (IGF-1; Smith et al. 2006). FAS gene is another target of the LXRs (Joseph et al. 2002; Hong et al. 2008).
The observation that incubation of the isolated SG in vitro reflects the relative rates of lipid biosynthesis as they occur in vivo, at least in their initial stage, has allowed studying metabolic pathways of sebogenesis (Downing et al. 1977). Experiments based on the incorporation of radiolabeled acetate, have demonstrated that the SG possesses the capacity to synthesize lipids de novo (Summerly and Woodbury 1971; Guy et al. 1999). Based on the patterns of lipids synthesized from different exogenous substrates, it has been suggested that NADPH was a limiting and directing factor of the sebaceous lipids biosynthesis in isolated SG (Middleton et al. 1988; Downie and Kealey 1998). Exogenous radiolabeled glucose, a good source of NADPH, favors TG synthesis, whereas exogenous radiolabeled acetate, a poor source of NADPH, is in favor of squalene. TG synthesis, unlike squalene synthesis, is heavily NADPH dependent (Middleton et al. 1988). This has suggested that the glandular level of NADPH is the limiting and driving factor leading to the squalene/TG ratio typical of sebum. Thus, sources of NADPH, such as glucose and glutamine, favor TG synthesis. Supplying sebocytes with acetate, which is a poor source of NADPH, results in a diminished abundance of TG, whereas squalene biosynthesis is favored. The synthesis of cholesterol from squalene is also NADPH dependent, raising the question whether the high amount of squalene in sebum would be a NADPH driven process (Ridden et al. 1990). Endogenous glycogen is a potential lipogenic substrate. Following glycogen breakdown, glycerophosphate is as important as NADPH in governing the sebaceous lipogenesis. Squalene synthesis is favored over TG when acetate and glutamine are the substrates, independently on the glycogen status of the gland (Downie and Kealey 1998).
Levels of Squalene in Skin Surface Lipids
Squalene accounts for 10–15 % of the total sebum lipids. (Kellum 1967; Downing et al. 1969, 1977; Greene et al. 1970; Stewart and Downing 1985). The SG has likely evolved a mechanism that halts the cholesterol biosynthesis and warrants the accumulation of squalene. On the other hand, the anaerobic environment of the SG might limit the access of molecular oxygen required for the further processing of squalene (Smith et al. 2008). The high content of squalene together with an exceptional variety and abundance of WE distinguishes the sebaceous lipid secretion from other lipid compartments. Noteworthy, elevated levels of squalene occur only in human sebaceous secretion (Nikkari 1974a). It can be speculated that squalene together with WE conveys the major skin protective purpose of sebum thought to be limitation of dehydration. The SG is fully functional at birth. The maternal androgens and the endogenous steroid synthesis stimulate the SG resulting in the secretion of the vernix caseosa, which appears starting from the third trimester of gestation. Composition of sebum has been evaluated from birth to adulthood. Although interindividual variations of squalene and other sebaceous lipids, reported as weight/weight percentage of skin surface lipids, is very high, average values show consistency in a wide range of ages (Table 12.1). Measures of sebum composition from infancy to adulthood demonstrated that varying the rate of sebaceous lipogenesis does not necessarily change the composition of the lipid mixture being synthesized (Table 12.1). In particular, squalene concentrations appear not to correlate with development from infancy to adulthood (Ramasastry et al. 1970). Upon elaboration of the early data on the sebum composition, it can be inferred that squalene and TG proportions are inversely correlated. This observation possibly indicates that factors regulating the squalene synthesis are negative modulators of TG (Table 12.1). This inverse relationship is consistent with the different dependency on NADPH of the respective biosynthetic pathways as discussed earlier (Middleton et al. 1988; Downie and Kealey 1998). Further evidence on the influence of substrate availability on the sebum composition arises from studies in humans. Changes in the concentration of sebaceous lipids on the skin surface in normal weight and obese human subjects resulting from fasting, demonstrated that squalene is the component that is preserved under caloric restriction. Starvation lowers the total sebum excretion rate and decreases the percentage of WE and TG. In contrast, the proportion of squalene increases upon starvation (Pochi et al. 1970; Downing et al. 1972). Thus, the reduction of sebum excretion occurring upon caloric restriction is due to relented synthesis of all sebum constituent except squalene. Estimate of the delay between the synthesis of sebum and its excretion to skin surface time came from the observation that the maximum rate of increase of squalene concentration in the skin surface lipids occurred at the eighth day after the fast was started. Together these evidences support the hypothesis that during caloric restriction or starvation the biosynthetic pathways leading to FA are shut down to preserve squalene biosynthesis. The authors concluded that alterations of nutritional status could be detectable by analyzing the skin surface lipids. The concentration of squalene in SSL is also indicative of the uneven distribution of the SG in different body sites. In particular, the squalene/cholesterol ratio is a useful marker of the proportion between sebaceous secretion and epidermal lipids. Forehead, chest, and back are in the order the body sites with the highest squalene/cholesterol ratio. Whereas lower values of squalene/cholesterol ratio were obtained from anatomical sites with less SG density, e.g., legs (Michael-Jubeli et al. 2011).
Table 12.1
Changes in surface lipid composition with age. (Modified from Ramasastry et al. (1970). Reported values are the weight/weight percentage of lipids in sebum)
Age range | Subjects | Free FA | TG | WE | Cholesterol | CE | Squalene |
---|---|---|---|---|---|---|---|
1 month–2 years | 7 | 20.8 | 38.4 | 17.6 | 3.7 | 10.3 | 9.4 |
2–4 years | 8 | 22.9 | 49.6 | 8.0 | 4.2 | 8.9 | 6.2 |
4–8 years | 13 | 15.9 | 45.6 | 6.9 | 7.2 | 14.6 | 7.7 |
8–10 years | 8 | 17.8 | 47.4 | 17.8 | 3.2 | 5.7 | 8.3 |
10–15 years | 7 | 18.8 | 42.9 | 23.6 | 1.8 | 4.2 | 8.4 |
18–40 years | 17 | 16.4 | 41.0 | 25.0 | 1.4 | 2.1 | 12.0 |
Correlations with squalene | − 0.5172 | − 0.7510 | 0.7707 | − 0.5746 | − 0.5597 | 1 |
Interaction Between Blood and Sebaceous Lipids
Squalene is measurable in blood, likely as a result of a lipid leakage from the cholesterol biosynthetic processes in metabolic tissues and from dietary sources (Rajaratnam et al. 1999). Squalene as the intermediate of cholesterol synthesis is included in surrogate markers of cholesterol metabolism and absorption. Early studies have demonstrated that squalene is accumulated in lipid bodies of human adipose cells (Tilvis et al. 1982). Significance of squalene stores in the adipose tissue has not been clarified. Shark liver oil is the richest source of squalene; however, there are several botanical sources as well, including rice bran, wheat germ, olive oil, palm oil, cottonseed oil, and rapeseed oil. Squalene is found abundant also in yeast lipid extracts. Squalene is absorbed from dietary sources, transported mostly in association with very-low-density lipoprotein (VLDL) and low-density lipoprotein (LDL), and distributed to the periphery (Simonen et al. 2007). Ingestion of squalene-rich dietary oils results into increased serum levels of squalene and conversion into cholesterol. (Strandberg et al. 1990; Miettinen and Vanhanen 1994). Serum concentration in healthy woman has been observed to range from about 1–3 μM and to be in correlation with cholesterol levels and the lipoprotein distribution. It remains to be elucidated how the blood levels of squalene are connected with the cholesterol absorption and metabolism through the conversion to lanosterol (Rajaratnam et al. 1999). The same group concluded that elevated blood levels of squalene were associated with higher risks of cardiovascular diseases in women. (Rajaratnam et al. 2000). Serum levels of squalene have been demonstrated to be associated with the prevalence of the metabolic syndrome (Gylling et al. 2007).
There is evidence that the activity of lipogenetic enzymes in the SG is regulated by exogenous lipids (Smythe et al. 1998). Nevertheless, the influence of circulating lipids on the lipogenic activity of the SG awaits further investigations. Cholesterol enters the cells by the LDL receptor-mediated endocytosis causing inhibition of HMG-CoA reductase transcription and translation and stimulation of the enzyme degradation (Goldstein and Brown 1990). As HMG-CoA reductase is regulated by the cholesterol levels in the SG environment, cholesterolemia could have some impact on the squalene levels in the sebum. Conflicting indications have been provided on the blood–skin interaction concerning cholesterol. In humans, 26–46 % of skin surface cholesterol is derived from the bloodstream (Nikkari et al. 1974b, 1975). However, the amount of skin cholesterol has no apparent relationship with hypercholesterolemic or normocholesterolemic conditions of subjects (Bhattacharyya et al. 1972). Nevertheless, the SG responds to higher levels of lipoproteins by lowering the lipidogenic capacity through inhibition of the HMG-CoA reductase activity; this evidence indicating intracellular delivery of exogenous cholesterol occurs through interaction with the LDL receptor (Smythe et al. 1998). Interestingly, the enzymatic activity of HMG-CoA reductase varies considerably in the apparently healthy skin of acne-unaffected individuals (CV = 30–46 %). Moreover, the declined sebum production occurring with age can be at least in part ascribable to the lower activity of HMG-CoA reductase in older subjects.
Functions of Squalene in Sebum
Multiple roles have been attributed to squalene, although the most specific and prominent one has not been recognized as yet. The typically high squalene concentration found in sebum is reached in fully differentiated and mature SG. As such, squalene is considered the biological marker of sebocyte differentiation. Altogether the SSL participate to the barrier function of skin in many respects. In particular, squalene covers several functions that range from the immunomodulatory, to the regulation of the skin microflora, to contrasting UV damages and skin ageing (Picardo et al. 1991a; Dennis and Shibamoto 1989; Chiba et al. 1999). As soon as the SG becomes fully active and excretes sebum, Proprionibacterium acnes (P. acnes) finds the favorable conditions for the colonization of the pilosebaceous follicle. With the limited knowledge accumulated so far, it has been concluded that P. acnes uses the sebaceous FA as nutrients upon hydrolysis of TG operated by its own lipases. Noteworthy, P. acnes colonization causes the incretion of photosensitizers, i.e., porphyrins, that participate in the photo-oxidative processes leading to lipid degradation, mainly affecting squalene integrity (Saint-Leger et al. 1986a). Alteration of SSL, both in quantity and quality, has been found to be involved in different skin diseases. Levels of SSL are affected in a number of skin disorders. Marked modifications of SSL have been reported in atopic dermatitis, psoriasis, and seborrheic dermatitis (Picardo et al. 1991b; Passi et al. 1991; Zouboulis 2010). The impact of these skin disorders on the SSL composition has been reviewed (De Luca and Valacchi 2010). Apart from compositional alterations of ceramides, which are pivotal in the homeaostasis of the skin barrier (Masukawa et al. 2008), subjects affected from atopic dermatitis present significantly lower levels of lipids of the sebaceous type, including squalene. Correspondingly, levels of other lipid species are increased, indicating that the lipid proportions in sebum are impaired in conditions of epidermal barrier disruption. Notably, systemic alterations of main lipophilic and enzymatic antioxidants are frequently associated with the above diseases (Passi et al. 1991).
In acne, about 30 % of the wet weight of follicular casts is formed by lipid matter, wherein squalene accounts for the 20 % of total lipids (Nordstrom et al. 1986). Although squalene has been addressed as a comedogenic factor, per se it is not an inflammatory mediator in acne, suggested by the observation that intradermal injection of squalene induces no inflammatory response in receiving subjects (Puhvel and Sakamoto 1977). In contrast, substances contained in sebum rather than squalene per se stimulate comedogenesis, providing the first clue that qualitative changes played a role (Kligman and Katz 1968). Sebum outflow is a route of excretion of lipophilic antioxidants to the skin surface. Vitamin E (α-tocopherol) and coenzyme Q10 are cosecreted with squalene in the skin surface lipids. Levels of α-tocopherol directly correlate with those of squalene, indicating a reciprocal influence in the mechanisms regulating their secretion and degradation by environmental factors (Thiele et al. 1999; De Luca and Valacchi 2010). The lipophilic antioxidants prevent squalene from excessive photooxidation, as they act as scavengers of free radicals and reactive oxygen species in the lipid phase (Passi et al. 2002). In spite of the low levels that exogenous antioxidants reach in sebum, they exhibit a synergic inhibition of the UV induced effects that results in the rescue of squalene depletion.
Squalene Oxidation Products and Skin Disorders
Squalene is regarded as the major lipid undergoing peroxidation in sebum, based on its chemical structure and its concentration in the SSL. Because of the elevated number of unsaturated sites, squalene represents a sensitive target of peroxidation reactions, wherein squalene monohydroperoxide is the primary oxidation product (Kohno et al. 1995). Due to the symmetry of the molecule, six possible positional isomers of monohydroperoxide derivatives are produced. Further oxidation of monohydroperoxides generates by-products bearing two or more –OOH groups (Mountfort et al. 2007). Oxidative reactions involving squalene yields other by-products, mainly squalene epoxides, also characterized by positional isomerism (De Luca et al. 1996; Mountfort et al. 2007). Furthermore, 2,3-squalene oxide can be formed enzymatically by mammalian or fungal squalene monoxigenases (Fig. 12.1). Several in vitro studies indicate that squalene oxidation can be initiated by light exposure and both UVA and UVB radiations. Squalene is very efficient in scavenging singlet oxygen, which is generated upon excitation of photosensitizing molecules, which enhance the yield and the kinetics of oxidation by-products formation (Ohsawa et al. 1984; Dennis and Shibamoto 1989; Kohno et al. 1995; Matsuo et al. 1983; Ryu et al. 2009). Breakdown products of oxidized squalene expand the chemical variety of the oxidation by-products. Transfarnesal and short chain reactive aldehydes, in particular formaldehyde and malonyl dialdehyde complete the wide range of by-products generated by oxidative degradation of squalene. (De Luca et al. 1996; Dennis and Shibamoto 1989; Yeo and Shibamoto 1992; Wei and Shibamoto 2007). It has been demonstrated that in physiological conditions, UVA induces oxidative degradation of squalene at a much higher extent than UVB (Ryu et al. 2009). Early reports of squalene peroxidation upon UVB were likely ascribable to the minimal UVA doses contaminating the UVB sources (Ekanayake Mudiyanselage et al. 2003). UV exposure of the skin produces marked squalene degradation especially when compared to the other unsaturated lipid fractions such as cholesterol, sebum TG, and free FA. Consistent with this, squalene can be considered as integral part of the SSL antioxidant defense providing skin lipids protection along with α-tocopherol and ubiquinone that are, at least in part, cosecreted and cosynthesized with squalene by the SG, respectively (Thiele et al. 1999; Packer and Valacchi 2002). However, it has been demonstrated that squalene oxidation takes place in vivo only after α-tocopherol and ubiquinone are depleted at a significant extent indicating that antioxidants are the first line targets of UV irradiation (Podda et al. 1998; Thiele et al. 2001; Shvedova et al. 2001). Squalene oxidation products generated by skin exposure to different peroxidative stimuli such as UV irradiation or microbial peroxidizing metabolism may be considered as important physiological mediators of the biological effects exerted on the skin by oxidative stimuli (Picardo et al. 1991c). For example, the discoloration observed in pityriasis versicolor, has been associated with the squalene oxidation (Nazzaro-Porro et al. 1986). Peroxidated squalene induces wrinkles and roughness in hairless mice, indicating that it partly delivers the pro-ageing effects of UV light (Chiba et al. 1999). Early studies have suggested that by-products of squalene oxidation are involved in the onset and progression of acne vulgaris (Leyden 1995). The lipid peroxidation hypothesis of acne pathogenesis has been unraveled in several studies revealing that localized free radical damage and peroxides might be involved in initiating the inflammatory reactions. Lipid peroxidation is evident in acne, wherein localized free radical damage and peroxides might be involved in initiating the damaging inflammatory reactions. Particularly, squalene shows enhanced comedogenicity when oxidized (Mills et al. 1978). Both squalene and its oxidized metabolites have been found at much higher levels in acne vs. healthy controls (Mills et al. 1978; Saint-Leger et al. 1986a , b; Hanaoka et al. 1971; Cotterill et al. 1972; Pappas et al. 2009). The mechanisms of squalene oxidation by-products formation in acne remain to be elucidated. Nevertheless, they are one of the factors of the pathological functions exerted by sebum. P. acnes metabolism produces coproporphyrin which has been demonstrated to be an efficient singlet oxygen generator upon UVA irradiation. This observation could provide a link between P. acnes colonization and increased oxidative damages of SSL in acne (Arakane et al. 1996). Application of UV-irradiated squalene onto cultured keratinocytes elicits proinflammatory effects, partly mediated by the increased expression of inflammatory cytokines and cell hyperproliferation (Ottaviani et al. 2006). Thus, inflammatory manifestations in acne are likely secondary events triggered by peroxidated lipids. It has been observed that the accumulation of lipoperoxides in the comedones correlates with the severity of the lesions and biomarkers of inflammation and cell hyperproliferation. Higher levels of lipid peroxides and IL-1α within the comedones were associated with the obstruction of the infundibulum observed at different stages of comedones formation, from microcomedones to inflammatory open or close comedones (Tochio et al. 2009). Specifically, by-products of squalene derived from oxidative processes have been related to comedones formation. The topical application of squalene monohydroperoxide has been proven comedogenic in animal models (Motoyoshi 1983; Chiba et al. 2000). In addition, the study of intra-comedonal lipids of acne patients indicated the presence of high amount of polar lipids that appeared mainly derived from squalene peroxidation (Saint-Leger et al. 1986b). Further mechanisms through which oxidized squalene causes detrimental effects are centered on the depletion of antioxidant defense systems (Ikeno et al. 2011). In vitro study on fibroblast demonstrated that glutathione is an important endogenous antioxidant implicated in the protection of skin cells from oxidative damage induced by squalene monohydroperoxide. Increased sensitivity to squalene peroxides along with a more pronounced comedogenic response have been observed upon glutathione depletion (Chiba et al. 2001). The pathogenetic role of squalene peroxidation in acne points out to antioxidants as suitable means to attenuate comedogenicity (Bowe et al. 2012). In particular, evidence has been provided on the value of oral vitamins A and E in attenuating lipid peroxidation in acne (Ayres and Mihan 1978).
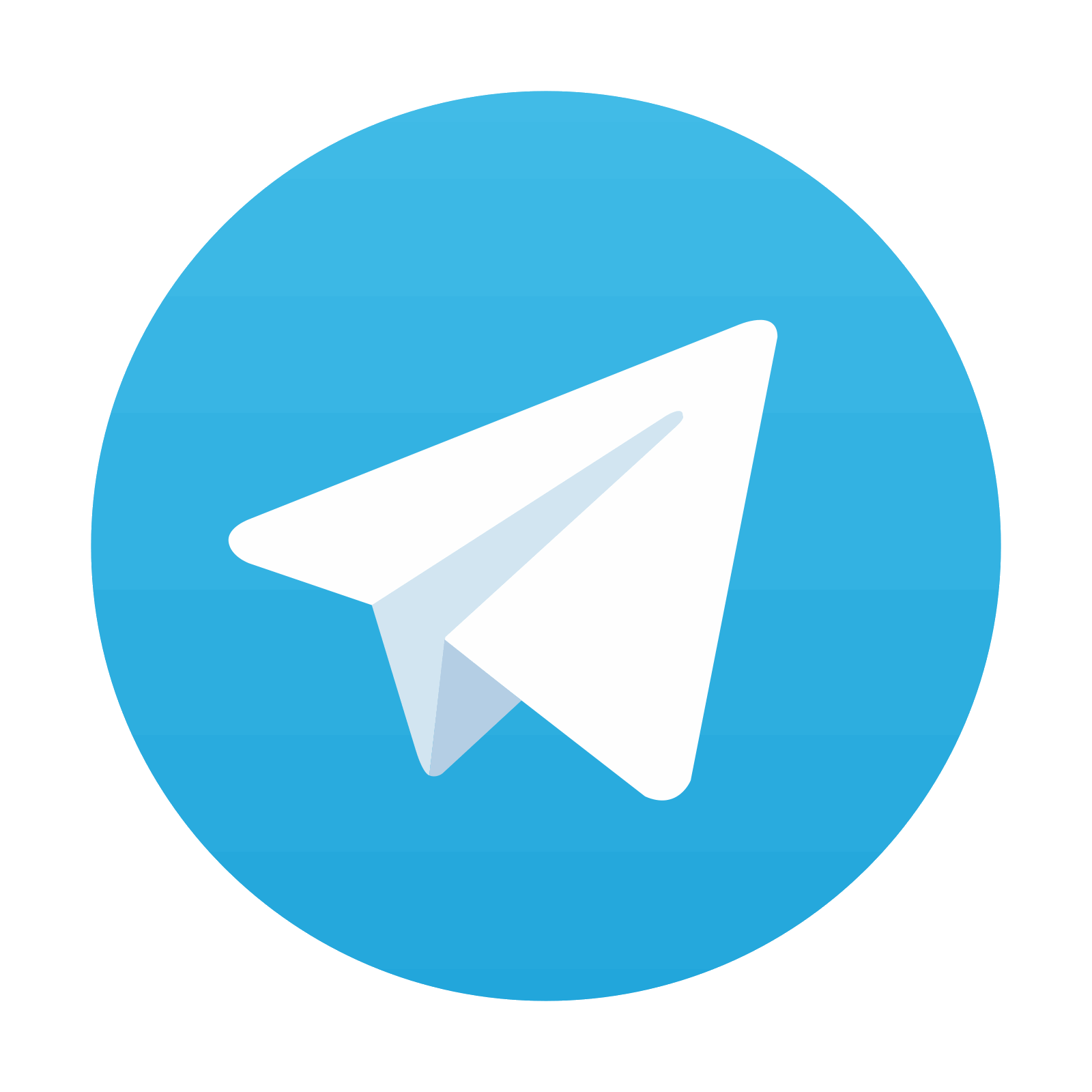
Stay updated, free articles. Join our Telegram channel
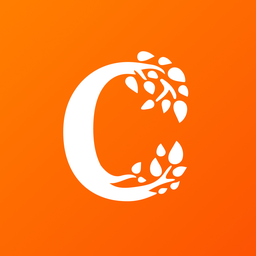
Full access? Get Clinical Tree
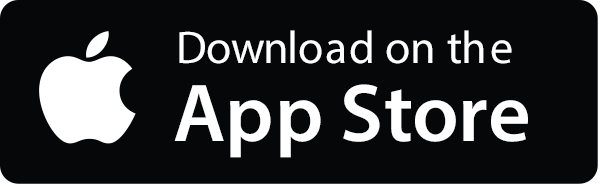
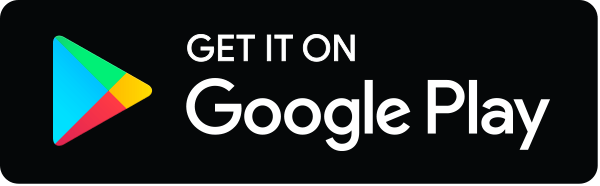