Traditional resurfacing techniques have their put period after drawbacks.
Traditional ablative resurfacing techniques and non-ablative laser and light procedures are well-established treatment modalities for modifying the appearance and characteristics of skin.1–3 However, patients have become hesitant to accept the side effects and risks associated with traditional ablative techniques.4 The main concerns of ablative resurfacing are prolonged procedural downtime, risk of scarring, and the incidence of delayed, long-lasting hypopigmentation, also known as ‘alabaster skin’.5,6 Further, traditional non-ablative techniques for collagen remodeling have shown inconsistent and often unpredictable results, frequently leading to unsatisfied patients and treating physicians.7 For a detailed description of the advantages and disadvantages of traditional resurfacing techniques, see Chaps. 9, 12, and 13 of this volume.
Fractional photothermolysis’ (FP) is a relatively new concept introduced by Manstein et al. in 2004. |
FP is based on generating patterns of microscopically small lesions, also called ‘microscopic treatment zones’ (MTZs). |
FP has become an established treatment –technique in dermatological laser therapy. |
To overcome the drawbacks of these traditional resurfacing approaches, fractional photothermolysis (FP) was developed by Manstein et al.8 In contrast to traditional laser techniques, which provide laser exposure to the entire skin surface, FP creates a pattern of microscopically small, 3-dimensional treatment zones (microscopic treatment zones or MTZs) while leaving the skin surrounding each of these MTZs substantially undamaged (Fig. 1). The term “fractional photothermolysis” was selected to express that only a fraction (lat: frangere, to break into pieces) of the skin is exposed to light (photo) to thermally damage or destroy (thermolysis) small treatment zones. FP procedures have been demonstrated to successfully treat a wide variety of aesthetic and dermatological indications. There is a broad range of possible treatment settings that may be used to generate the resulting wound healing response. FP can be delivered in multiple treatments, each providing incremental improvement within the limits of individual patient’s tolerance for downtime and side effects. Therefore FP gives the physician additional options to provide a customized treatment regime that is suited to fit their patient’s needs and expectations. FP procedures have become increasingly popular over the past few years and have become an established concept in dermatological laser therapy. The rapid proliferation of publications in the dermatological literature related to FP is an indication of the significant impact that FP had on the field (Fig. 2). The success of FP is also documented by the wide variety of commercially available FP devices; virtually all dermatological laser device companies currently have at least one FP device in their product portfolio (Tables 1 and 2).
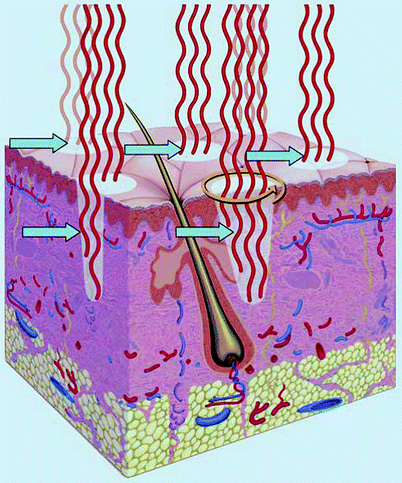
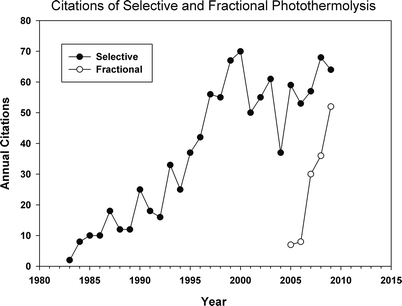
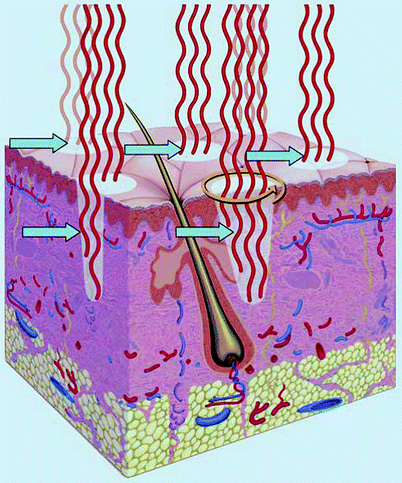
Fig. 1
Concept of fractional photothermolysis. An array of microscopically small zones of thermal injury, so called MTZs (microscopic treatment zones, gray arrows), is generated by multiple, focused laser pulses. Each of the MTZ is surrounded by undamaged skin (orange, circular arrow), allowing for fast repair (Laubach and Manstein9)
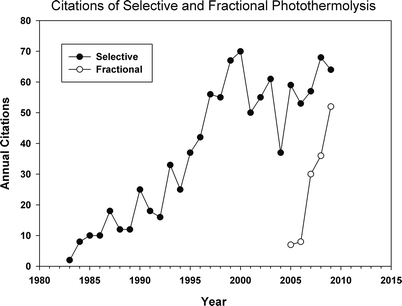
Fig. 2
Annual citations of the original article for fractional photothermolysis (FP) and selective photothermolysis (SP). Data obtained from ‘ISI Web of KnowledgeTM’, Thompson Reuters. The rapid proliferation of FP related publications indicates an increasing impact of this concept in the field of dermatology. The annual citations of FP have already reached a level comparable to that of SP. Further analysis reveals that recently SP citations are increasingly for non-dermatological indications, e.g., in ophthalmology or otorhinolaryngology. In contrast, FP citations are so far generally limited to dermatological indications (SP: Anderson and Parrish10; FP: Manstein et al.8)
Table 1
Characteristics of selected, commercially available non-ablative fractional photothermolysis (nFP) devices, including manufacturer, device name, laser type, emitted wavelength, nominal spot size and range of energy/MTZ. This table is not comprehensive, the pulse duration listed device parameters were obtained by manufacturer survey and are also subject to change.
Company | Device | Laser | Wavelength [nm] | Delivery | Spot size [μm/MTZ] | Energy [mJ/MTZ] | Pulse duration [ms] |
---|---|---|---|---|---|---|---|
Cynosure | Affirm | Nd:YAG | 1320 / 1440 | Stamping | 100 – 150 | up to 4.1 | 1, 1.5, 2 |
Lutronic | Mosaic | Er:Glass | 1550 | Scanned stamping | 4 – 40 | 0.5 – 4.5 | |
Palomar | Lux1540 | Er:Glass | 1540 | Stamping | up to 100 | 5, 10 | |
Quanta | Matisse | Er:Glass | 1540 | Stamping | up to 20 | 4 – 14 | |
Sellas | 1550 | Er:Glass | 1550 | Scanned stamping | 312 – 1000 | 1 – 30 | 0.5 |
Solta | Fraxel re:fine | Er:Glass | 1410 | Rolling | 5 – 20 | ||
Solta | Fraxel re:store DUAL | Er:Glass | 1550 / 1927 | Rolling | 135 / up to 600 | 4 – 70, 5 – 20 | 0.015 – 3 |
Table 2
Characteristics of selected, commercially available ablative fractional photothermolysis (FP) devices, including manufacturer, device name, laser type, emitted wavelength, average optical emission power, nominal spot size and range of energy/MTZ. This table is not comprehensive, the pulse duration listed device parameters were obtained by manufacturer survey and are also subject to change.
Company | Device | Laser | Wavelength [nm] | Delivery | Power [W] | Spot size [μm/MTZ] | Energy [mJ/MTZ] | Pulse duration [ms] |
---|---|---|---|---|---|---|---|---|
Alma | Pixel CO2 | CO2 | 10600 | Scanned stamping | 40, 70 | 250 | 50 – 300 | |
AMT | Touch Cell | CO2 | 10600 | Scanned stamping | 30 | 200 | ||
Candela | QuadraLase | CO2 | 10600 | Scanned stamping | 20 | |||
Cutera | Pearl Fractional | YSGG | 2790 | Scanned stamping | 300 | 60 – 320 | 0.6 | |
Cynosure | Smart Skin | CO2 | 10600 | Scanned stamping | ||||
Deka | SmartXide DOT HP | CO2 | 10600 | Scanned stamping | 50 | 300 | 0.2 – 80 | |
Ellipse | Juvia | CO2 | 10600 | Scanned stamping | 10 | 500 | 5 – 15 | 5 – 7 |
Lasering | Mixto | CO2 | 10600 | Scanned stamping | 20 | 180, 300 | 2.5 – 16 | |
Lumenis | Active FX | CO2 | 10600 | Scanned stamping | 60 | 1300 | 5 – 225 | 0.04 – 2 |
Lumenis | DeepFX | CO2 | 10600 | Scanned stamping | 60 | 120 | 5 – 50 | 0.04 – 0.2 |
Lutronic | eCO2 | CO2 | 10600 | Scanned stamping | 30 | 120,180,300 | 4 – 120 | |
Palomar | Lux2940 | Er:YAG | 2940 | Stamping | 80 – 125 | up to 25 | ||
Quanta | youlaser CO2 | CO2 | 10600 | Scanned stamping | 30 | 0.05 – 20 | ||
Quantel | BuraneFX | Er:YAG | 2940 | Scanned stamping | 150 | 0.7 – 32 | 0.35 – 250 | |
Quantel | ExelO2 | CO2 | 10600 | Scanned stamping | 40 | 350 | 10 – 1000 | 1 – 100 |
Sciton | Profactional | Er:YAG | 2940 | Scanned stamping | 250 | |||
Solta | Fraxel re:pair | CO2 | 10600 | Scanned stamping | 40 | 135, 600 | 5 – 70 | 0.15 – 3 |
Individual MTZs are typically so small that they induce neither extended inflammation nor fibrosis. |
MTZs heal quickly due to their small cross section and the presence of adjacent unharmed tissue. |
MTZs can extend down to the deep reticular dermis for certain laser parameters. |
One of the key concepts of FP is that the individual damaged tissue regions (MTZs) are so small in at least one dimension that the damaged or destroyed tissue is rapidly repaired without any significant fibrosis. The close proximity of surrounding viable tissue facilitates the wound healing. Although the individual MTZs induce a guaranteed wound healing response, the extent of inflammation is limited and confined to their close proximity. The overall wound healing response is primarily determined by the shape of individual MTZs and their distribution (density) within the treatment area. This “fractional” approach contrasts with thermal wounds having larger dimensions and longer healing times, which are typically generated using conventional resurfacing procedures or the like. It remains a subject of further research to define the particular conditions favoring tissue repair over fibrosis. Another advantage of generating lesions on a microscopic scale is that the individual lesions are so small that typically they cannot be resolved with the naked eye under clinical conditions, thereby ensuring a homogenous appearance of the treated area. MTZs heal quickly due to their small cross section and the presence of unharmed tissue. They can extend down to the deep reticular dermis and still be well-tolerated in terms of rapid healing. Such deep tissue destruction has to be carefully avoided with traditional ablative resurfacing techniques, as confluent damage to such depth levels would impair the skin’s ability to regenerate, and would most likely result in scarring similar to that seen in third-degree burns.11
Two distinct types of FP exist: non-ablative FP (nFP) and ablative FP (aFP). |
Non-ablative FP (nFP) generates MTZs characterized by thermal coagulation. |
Ablative FP (aFP) generates MTZs exhibiting some degree of immediate tissue removal (vaporization) and a surrounding zone of coagulated tissue. |
A distinction between superficial, medium and deep FP procedures may facilitate description of the procedure, but is somewhat arbitrary. |
At present, two distinct types of FP exist – non-ablative FP (nFP) and ablative FP (aFP) (Fig. 3). Non-ablative FP (nFP) generates MTZs having a small-diameter zone of thermally damaged epidermis and dermis extending down to a particular depth. The shape of such MTZs is either an inverted cone or a tapered column extending into the dermis. The degree of thermal damage within an MTZ is typically sufficient to cause cell necrosis and to coagulate collagen. The physical integrity of the skin remains intact, in spite of the marked localized thermal damage.8 Ablative FP (aFP), on the other hand, creates MTZs by vaporizing microscopic zones of tissue up to a particular depth. This depth is primarily dependent on pulse energy and may extent into the deep reticular dermis. The resulting tapered cavity is lined by a thin layer of eschar and surrounded by a cuff of thermal denaturation, which is sufficient to destroy cells and coagulate collagen. Ablative FP results in immediate tissue loss due to the physical removal of portions of the skin by vaporization, and the physical integrity and barrier function of the skin is locally compromised.12 As the depth of MTZ can vary greatly, it appears reasonable to further distinguish between FP procedures generating MTZs of different depths, e.g., superficial, medium and deep. While such a classification may serve to better describe a FP process, classification by damage depth is somewhat arbitrary. It remains to be determined how the depth of MTZs affects the clinical outcome for various FP protocols.
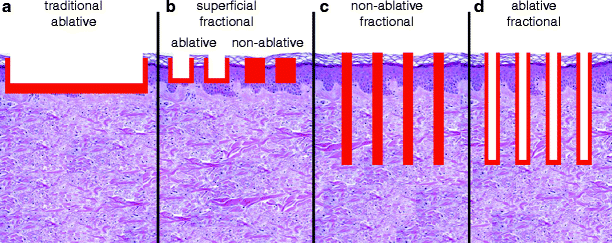
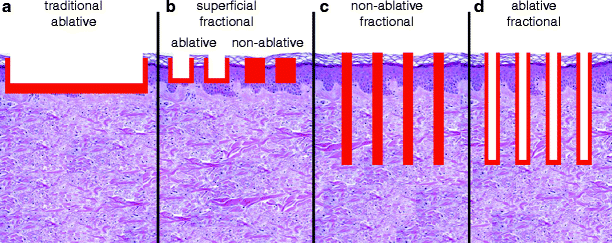
Fig. 3
Schematics of different resurfacing modalities. Traditional ablative procedures generate a confluent layer of tissue removal and with thermal coagulation to the adjacent remaining tissue (a). Mainly, two distinct types of FP procedures exist, ablative (aFP) and non-ablative (nFP) photothermolysis. nFP creates multiple microscopic treatment zones (MTZs) consisting of coagulated tissue. Despite of the thermal damage, the physical integrity of the skin remains intact (c). AFP vaporizes some tissue, creating multiple MTZs which consist of a cavity lined by a thin layer of eschar and surrounded by a cuff of coagulated tissue (d). Further differentiation of FP procedures according to the depth of individual MTZ is possible, e.g., superficial FP, but somehow arbitrary (b)
The laser wavelength determines primarily if an FP procedure is ablative or non-ablative. |
Ablative FP procedures are performed with lasers emitting at wavelengths corresponding to strong absorption bands of water. |
The depth of an MTZ for nFP is limited by the optical penetration of the particular wavelength into skin tissue. |
FP procedures generate a 3-dimensional pattern of MTZs in the skin and the wound healing response is primarily determined by the characteristics of individual MTZs and their spatial distribution. The laser tissue effects generated within individual MTZs depend primarily on applied wavelength, pulse energy, focused beam diameter and pulse duration.
The laser wavelength plays an important role in the characteristics and results of an FP procedure. The main chromophore absorbing the laser energy, either for nFP or aFP, is water. Wavelengths that are very strongly absorbed can result in local volumetric energy densities sufficient to vaporize tissue.13 Therefore aFP procedures are performed with lasers emitting at wavelengths corresponding to strong absorption bands of water. The approximate optical penetration depth (OPD) in water for such lasers is minimal, e.g., 1 μm for the Er:YAG laser (λ = 2,940 nm) and 10 μm for the CO2 laser (λ = 10,600 nm).14 High volumetric energy densities are reached virtually instantaneously within the focus of the laser beam, and therefore such lasers can quickly advance a cavity deep into the tissue during the pulse. Due to this process it is possible that the resulting depth of an MTZ can greatly exceed the optical penetration depth of any particular laser wavelength. Once the local energy density exceeds the vaporization threshold, the depth of the laser created cavity is primarily related to the total energy delivered for a given spot size, and relatively independent of the applied wavelength. It should be noted, that the Er:YAG typically produces less thermal damage in the residual tissue as compared to the CO2 laser due to the stronger absorption by water.
As nFP procedures do not physically remove tissue, the maximum depth of MTZs in nFP procedures is limited by the optical penetration depth of any particular laser wavelength. Variation of the applied laser energy allows some adjustment of the thermal injury depth within this physically imposed limit. However, several points should be considered when applying this concept to real procedures. The optical penetration depths provided are approximations of the penetration depth in water provided by Hall.14 The water content of tissue can vary substantially and is approximately 30% for the epidermis and 70% for the dermis.15 As the optical properties of water are also temperature dependent, it has been reported that the rapid change of tissue temperature during a laser pulse can dynamically alter the penetration depth substantially.16 Also, other factors such as scattering, phase transitions (e.g., collagen denaturation), and non-linear phenomena should be taken into consideration for a more detailed analysis of the wavelength dependent effects on the shape of MTZs. The following are examples of lasers that can be used for nFP, together with their respective wavelengths (λ) and approximate optical penetration depth (OPD): Er:YAG (λ = 1,440 nm, OPD ≈ 300 μm) Er:Glass (λ = 1,540 and 1,550 nm, OPD ≈ 1,000 μm), and Thulium fiber laser (λ = 1,927 nm, OPD ≈ 100 μm). These differences in optical penetration lengths indicate why the Thulium laser, with a relatively shallow penetration depth, is often used to treat superficial lesions within the epidermis and papillary dermis, and why the Er:Glass laser with a relatively larger optical penetration depth can generate MTZs extending down into the mid to deep reticular dermis. Because these provided numbers are estimates that which can be affected by various factors, they should serve only as a general guideline, and not as a specific reference.
The pulse duration and temporal pulse profile can affect the amount of thermal damage in the surrounding tissue. |
The pulse duration for FP systems is typically in the range of up to a few milliseconds, but varies with the preset energy applied per MTZ. The MTZ energy is controlled for most FP systems by adjustment of the pulse duration used to create individual MTZs. In a first approximation, such short pulse durations are within the thermal relaxation time of individual MTZs and minor variation of pulse duration should have limited effects on lesion shape. However, variation of pulse duration over an extended range of pulse profiles will affect the MTZ shape, e.g., ablation depth and/or extent of residual thermal damage. Tissue effects related to pulse duration and/or temporal pulse profile have not yet been characterized in detail, but these parameters are likely to be the focus of future studies for optimizing FP procedures. The available average power of an FP laser system is a critical factor that limits the maximum overall coverage rate. For example, a laser that delivers a higher average optical power can be capable of faster treatment of larger areas.
Coverage of a treatment area can be achieved by the ‘stamping’ or ‘rolling’ technique. |
Typically, multiple passes are necessary to provide sufficient treatment coverage. |
Variation of the number of passes and exposure settings in different areas can be used to adjust the clinical outcome in specific locations, e.g., ‘feathering’ at the edges of the treatment area. |
Excessively high treatment densities should be avoided as they can result in undesirable side effects. |
There are two general techniques currently available for generating the desired density of MTZs (number per unit area) within the treatment area: the ‘stamping’ technique and the ‘rolling’ technique. The ‘stamping’ technique is performed by forming a preset pattern of multiple MTZs on a skin region within a well-defined exposure area of the fixed handpiece, and then moving the handpiece to another skin region and repeating until the entire treatment area is covered. The density of MTZs at the end of a treatment session depends on the preset density within the exposure area of the handpiece and the number of passes performed over each skin region. A pass is defined as the coverage resulting from a single application of the handpiece to a particular area of the skin. The ‘rolling’ technique is performed by continuously rolling the handpiece across the entire treatment area. It is also referred as ‘brushing’ technique, because the movements of the operator are similar to using a paint brush. As the velocity of the handpiece relative to the skin varies during treatment, the delivery rate is adjusted automatically in order to maintain a defined, preset MTZ density per pass. The total density of MTZs at the end of a treatment session can be estimated as the density of MTZs per pass multiplied by the number of passes performed. However this presents only an estimate as with each pass the remaining undamaged skin surface decreases and therefore the effective amount of tissue that is newly damaged with each subsequent pass decreases. Some MTZs formed on subsequent passes may overlap or coincide with MTZs already formed during prior passes. A more detailed description of the determination of coverage in terms to number of passes is provided by Manstein et al.17 There appears to be no single best technique for delivering the desired density of MTZs. The ‘rolling’ technique can facilitate treatment of larger areas, while the ‘stamping’ technique can facilitate the precise treatment of smaller areas, in particular areas having an irregular surface profile. It is the opinion of the authors that a reasonably well-defined MTZ density can be achieved with both techniques, and the choice between stamping and brushing ultimately comes down to a personal preference of the operator. FP systems generally allow the operator to adjust both MTZ density and MTZ characteristics independently within the treatment area. The MTZ density can be adjusted by varying the preset number of pulses per area and/or number of passes, while the dimensions of individual MTZs can be modified by adjustment of the MTZ energy, energy beam focal characteristics, etc. Such control allows, for example, formation of a decreased MTZ density at the periphery of a treatment area to avoid demarcation lines between treated and untreated areas (feathering), or an increased MTZ density or applied energy density within particular areas that can benefit from an enhanced treatment outcome. The overall extent of the wound healing response, and thus the extent of both clinical improvement and side-effects appear to be related to the total amount of thermal injury or total energy delivered per treatment area. Treatment densities that result in confluent thermal injury can result in blistering or even scarring.
Bulk heating is the temperature rise of the tissue between individual MTZs by thermal conduction as each MTZ acts as a local heat source. |
Limited bulk heating may be desirable in FP procedures. |
Excessive bulk heating can result in confluent tissue damage and severe side effects including scarring. |
Excessive bulk heating can be avoided by limiting MTZ densities, extending the time interval between passes and application of cooling. |
Changes in tissue temperature effect the shape of individual MTZs. |
While FP procedures are designed to deliver localized thermal injury within individual MTZs, it should be taken in consideration that energy deposited into the tissue may accumulate under certain conditions. The temperature gradient tends to be very high within a single MTZ, often resulting in a very sharp demarcation between coagulated and non coagulated collagen. However, each MTZ represents a small heat source within the surrounding tissue. This heating effect has two principal consequences.
First, although there is typically a very sharp demarcation at the perimeter of an individual MTZ, thermal effects on the tissue immediately surrounding an MTZ have been observed. For example, apoptotic cell death and induction of various heat shock proteins can be seen close to individual MTZs. Although the effects of such events on the clinical outcome have to be further investigated, it can be speculated, that up to a certain extent, these local heating effects around the MTZs may enhance wound healing and clinical outcome.
Second, as each of the MTZs acts as a local heat source, forming many MTZs within a short time period can lead to an increase in the average tissue temperature of the treated region due to heat conduction. Such tissue heating is described by the term ‘bulk tissue heating.’ Bulk tissue heating can become a significant problem when the local average tissue temperature rises above a critical temperature such that confluent areas of tissue are damaged or destroyed, rather than limiting such damage to discrete small microscopic zones. Such gross thermal injury mimics that of a third degree burn, which can lead to substantial side effects including scarring.
The following precautions should be taken into consideration in order to avoid excessive bulk heating during FP procedures:
(a)
For higher individual MTZ formation energies, the spatial density of MTZs formed in the treatment region should be decreased.
(b)
When multiple passes are performed on a treatment region, the time interval between passes should be long enough to allow the tissue to cool down between consecutive passes.
(c)
External cooling, e.g., forced air cooling, can be used to remove some heat from the tissue region being treated.
Changes in tissue temperature have been shown to affect the geometry of individual lesions. Reduction of MTZ dimension due to decrease of tissue temperature have been shown to be more marked for nFP18 as compared to aFP.19 Skin cooling before, during and/or after FP treatments is often desirable because it can alleviate pain during treatment and also reduce the risk of bulk heating. Because skin cooling can also decrease the MTZ lesion size resulting from particular system settings during an nFP procedure, it is important to perform such procedures under standardized cooling conditions to control the thermal damage within individual MTZs. It should be noted that during aFP procedures, a substantial part of the laser energy is removed from the tissue with the hot laser plume. This is in contrast to nFP procedures, and therefore it is reasonable to conclude that for the same applied energy per MTZ energy and MTZ density, the overall (bulk) heating of tissue is greater for nFP as compared to aFP. Although, no studies have been carried out to either confirm or quantify this effect, the operator should be aware of such potential interrelated effects that are based on principles of laser tissue interaction.
FP procedures are typically performed as multiple treatments. |
Treatment outcome is typically incrementally enhanced after additional FP treatments. |
Typically, a series of approximately 3–5 nFP or 1–3 aFP treatments are performed. |
There is some controversies regarding whether the clinical improvement of an aggressive treatment can be achieved by repetition of less aggressive treatments. |
FP treatments allow distribution of the thermal wounding resulting from individual MTZs at different densities and over distinct treatment sessions. |
FP treatment of a particular skin region can be can be delivered in single or multiple treatment sessions. Typically 3–5 nFP or 1–3 aFP treatment sessions are performed, but the number of treatments can vary within a wider range depending on indications, treatment settings and patient response. Each treatment is customized to a patient’s individual condition to best manage side effects and downtime. The treatment is repeated until either the desired outcome is achieved or no further relevant improvement can be achieved. It should be remembered that some of the effects, e.g., collagen remodeling, can progress over a period of weeks or months after a treatment. Multiple treatments are generally performed at intervals of approximately 4–8 weeks. However there are no studies currently known that compare the outcomes achieved based on variation in treatment intervals. The current intervals of several weeks are generally preferred because they allow sufficient time for side effects to subside and also arguably because such intervals are convenient for the appointment scheduling of most offices and patients.
There is some controversy regarding the effect of multiple treatments sessions on enhancement of treatment outcomes. While it is generally accepted that multiple treatments sessions can improve the overall outcome, it is not clear exactly how the number of treatments sessions is related to the overall improvement. Also, it is still not known whether multiple treatments performed at well-tolerated settings can mimic the outcome of fewer or single treatments sessions performed with more aggressive settings that are associated with a marked wound healing response and prolonged downtime. FP treatments provide the possibility of obtaining particular degree of thermal wounding within individual MTZs and varying just the density of such MTZs and/or adjusting the number of sessions. In contrast, conventional treatments that cover the entire treatment area continuously do not allow for this freedom. These full-surface procedures only allow for adjustment of the treatment level by varying the fluence applied over the entire area.
Several factors beyond the laser parameter settings can affect the thermal damage. |
Mechanical manipulation of the skin such as compression, stretching and contraction can affect the shape and density of MTZs. |
A variety of factors beyond an FP system’s preset MTZ exposure/energy and density settings can affect the thermal injury of the tissue. The operator should be aware of such factors, as they can impact the wound healing response, clinical outcome, and side effects experienced by the patient. For example, mechanical factors such as tissue stretching, contraction, or compression can affect MTZ lesion dimension and MTZ density. Stretching of the skin during exposure can lead to an increased actual density of MTZs. The density per pass is typically preset by the system for a fixed exposure area, but skin stretching during the exposure can actually result in relatively higher MTZ density. This can occur because as the skin is able to retract after the stretching is relieved, any number of delivered pulses is located within a relatively smaller area. MTZs of smaller cross section can also result from stretching of the skin prior to treatment, as the MTZs that are formed in the stretched skin may shrink in size when the skin is allowed to retract. Point compression can distort the skin dimensions locally during exposure, and relatively deeper MTZs with smaller cross sections can result from such mechanical tissue manipulation. Also, because skin may contract as a result of localized thermal injury to the collagen, the dimensions of the skin can change during the delivery of a series of passes to generate individual MTZ patterns. Ablative FP procedures performed, particularly when performed with higher MTZ energies, tend to exhibit such shrinkage of the tissue during multiple FP passes over a particular treatment area.
In addition to the shape and density of individual MTZs, the number of treatment sessions and intervals can be chosen by the operator. |
The broad variety of possible combinations allows tailoring patient treatment protocols to specific needs. |
The multivariate complexity of FP procedures represents a challenge to obtain comparative clinical data. |
Most FP treatments result in varying degrees of clinical improvement for appropriate indications. |
The three basic rules of any FP treatment are: |
A. Individual MTZs should induce wound healing but not fibrosis. |
B. Confluent damage and bulk heating should be avoided. |
C. The cumulative MTZ density should be sufficiently high to result in clinical improvement after the completion of a treatment course. |
As discussed herein, many factors can affect thermal damage patterns generated in the skin and subsequent wound healing responses. Such factors include laser exposure parameters (e.g., energy per MTZ and focal spot size), the number of passes and time interval between them, mechanical tissue manipulation, use of skin cooling procedures, and others. The treatment interval between individual passes within a single treatment session and the number of sessions can also be varied. This virtually unlimited number of possible treatment combinations provides the possibility of tailoring patient treatment protocols to specific needs. However, this flexibility also leads to some complexity and uncertainty associated with the choice and control of all possible parameters and factors. The multivariate complexity of FP procedures explains in part the current lack of clinical studies comparing the effect of many specific FP parameters on patient outcomes. In spite of this complexity, it turns out that most FP treatment regimes result in some kind of clinical improvement for appropriate indications. Also, the fundamental principles of FP that guide the selection of treatment parameters are relatively simple and can be summarized by three basic rules. First, the dimensions of individual MTZs should not exceed certain dimensions, such that the induced wound healing results in tissue repair rather than inducing fibrosis. Second, the overall density of MTZs should not be excessively high to maintain sufficient undamaged tissue between the MTZs and facilitate tissue repair. In particular, thermal damage to confluent tissue via bulk heating should be avoided. Third, the cumulative density of MTZs should be sufficiently high to induce sufficient clinical improvement after a completed course of FP treatments.
When the concept of FP was first introduced, the laser was used as the energy source to generate fractional damage to the skin. The laser is still the most common energy source used in FP procedures. Its ability to quickly deliver energy in the form of focused optical radiation with high precision into small confined zones makes the laser a modality well suited for FP. Recently, other energy sources have emerged for generating fractional damage patterns. For example, radiofrequency (RF) and ultrasound devices are now commercially available that generate a pattern of small and confined thermal damage zones in skin tissue. The shape and anatomical location of MTZs generated using such modalities typically differ from those induced by focused optical radiation because of a different energy distribution within the tissue. As RF energy quickly diverges with increasing distance from the delivering electrode, it is possible to generate a spatially confined RF generated thermal injury only within the tissue directly adjacent to the tip of a needle electrode. Depending on the location of the tip of such RF electrode, damage can be generated either at the skin surface,20 or virtually at any depth by inserting needle electrodes into the skin,21 The use of stamping techniques with arrays or linear arrangements of multiple needle electrodes allows for coverage of a treatment area within a reasonable time. Focused ultrasound non-invasive generation of confined lesions,22 in skin layers such as, e.g., the deep reticular dermis or even the superficial musculoaponeurotic system (SMAS) without causing any surface damage.23 The MTZ cross section of RF or ultrasound generated MTZs is typically larger than that of laser generated MTZs because laser radiation can be more focused. However the ability to focus optical radiation decreases with increasing skin depth due to scattering and absorption of optical radiation. Further investigations are needed to investigate how the size and location of thermal lesions generated using RF and ultrasound sources affect the clinical outcome as compared to laser-generated MTZs.
aFP and nFP can treat a wide variety of clinical conditions including some that have been traditionally the domain of selective photothermolysis (SP). |
The relative benefits and disadvantages of ablative and non-ablative FP approach are being investigated. |
Both aFP and nFP target water-containing tissue and, unlike selective photothermolysis procedures,10 there is no significant selectivity of specific components because virtually all cells of the skin are composed primarily of water. Nevertheless, FP can be used to treat certain conditions that traditionally have been a domain of selective photothermolysis, including treatment of pigmented and vascular lesions. FP targets aqueous tissue that contains such target lesions and therefore can affect a variety of lesions. Both, aFP and nFP have been applied successfully to a variety of clinical indications, including collagen remodeling and treatment of vascular and pigmented lesions. Further details of indications and the wound healing process are described in the following sections. The balance between improved clinical efficacy of aFP for selected indications as compared to nFP and the additional risks and side effects of aFP associated with a impaired epidermal barrier function and removal of entire columns of tissue in aFP is still being explored.