Fig. 14.1
Physiological wound healing. Wound healing involves a series of partly overlapping phases. Clot formation ensures a first sealing of the wound to prevent microbial invasion. Within a few hours after injury, first polymorphonuclear neutrophils (PMN) and later macrophages invade the wound site in the inflammatory phase of wound healing to further combat contaminating microorganisms and to phagocytose cellular debris. The late inflammatory phase with macrophages as the prevailing inflammatory cells is followed by granulation tissue formation with wound contraction and angiogenesis and the matrix remodeling phase. The arrow indicates that in chronic wounds, the inflammatory phase of wound healing is prolonged and often persists, leading to chronic wounds which fail to progress through the pattern of normal healing
Aging occurs on a molecular, cellular, tissue, organ, and organismal level and involves a variety of distinct cell types, among them MSCs. Mitochondrial dysfunction with increased levels of reactive oxygen species (ROS) contributes to DNA damage accumulation, telomere dysfunction, and perturbed proteostasis observed in aged skin with the activation of distinct cellular check point responses such as apoptosis, replicative senescence, differentiation, repressed self-renewal, and the adoption of a senescence-associated secretory phenotype (SASP) eventually leading to the overall decline of tissue and organ homeostasis, repair, and regeneration. Currently, the contribution of intrinsic MSC aging to skin aging and impaired tissue repair is not well understood, and most of the studies on MSC aging are relying on in vitro evidence. This is mainly due to the lack of a MSC-specific marker, which would allow tracing individual MSCs in situ in skin and other organs. Nevertheless, the rare and specialized MSCs are supposed to be essential for the maintenance of cutaneous homeostasis and integrity (wound repair) throughout life. With advancing age, the number of MSCs and possibly their function decline with the observed overall compromised potential for regeneration and repair of damaged cells and tissues.
In this chapter, we present an overview of the physiological and therapeutic roles of MSCs on cutaneous wound repair and attempt to highlight the impact of age-induced changes in MSCs affecting their capacity for wound healing and scar remodeling. We conclude with a perspective for clinical applications with MSC-based treatment for age-associated wound-healing defects.
14.2 Mesenchymal Stem Cells in Skin Wound Repair
The focus of dermatological stem cell research is predominantly on the epidermis and the hair follicle. In contrast, the characterization of stem cells in the mesenchymal compartments of the skin has largely escaped the attention of the dermatologic community, though the dermis represents a larger reservoir for adult stem cells than the epidermis and the hair follicle together (Sellheyer and Krahl 2010). Currently, no in situ aging data on MSC populations are available. Nevertheless, MSCs have already shown great therapeutic promise for the treatment of age-related chronic wounds in the recent decade. Therefore, this review will preferentially focus on functions and cellular mechanisms of MSCs in the repair of chronic wounds.
14.2.1 Physiological Localization of Dermal MSCs in the Skin and Their Function During Wound Healing
In 2001, MSCs residing within the dermis were first isolated. They are endowed with the capacity to differentiate into adipocytes, smooth muscle cells, osteocytes, chondrocytes, neurons and glia, and hematopoietic cells of myeloid and erythroid lineages (Toma et al. 2001). The perifollicular connective tissue sheath and the dermal papilla represent the likely anatomical niche for these multipotent dermal cells, a finding proven in animal models (Jahoda et al. 2003; Hoogduijn et al. 2006). The primary adult hair follicle dermal papilla and dermal sheath MSCs revealed a fibroblastic morphology and were CD44+, CD73+, and CD90+. These MSC subpopulations were capable of dye exclusion suggesting a multidrug resistance property and had the capacity to differentiate into various mesenchymal lineages, such as osteoblasts, adipocytes, chondrocytes, myocytes, and expressed neuroprogenitor cell markers (Jahoda et al. 2003; Hoogduijn et al. 2006). Subsequently, MSC-like cells have been isolated from human dermis by other investigators (Bartsch et al. 2005; Lorenz et al. 2008; Vaculik et al. 2012); however, in these studies the detailed in situ localization of the reported MSC subpopulations was not illustrated.
During anagen hair development, connective tissue sheath and dermal papilla are not only remodeled but also impressively expanded (Tobin et al. 2003). This strongly suggests the presence of resident mesenchymal precursor cell populations. During each anagen hair development, angiogenesis occurs, which likely arises from mesenchymal stem cells residing in the connective tissue sheath (Jahoda et al. 2003). Moreover, the follicular connective tissue sheath serves as a crucial cell pool for the formation of granulation tissue, which is formed by fibroblasts, endothelial cells, mast cells, and macrophages, after substantial skin trauma (Jahoda and Reynolds 2001).
Besides the skin-resident dermal MSCs, the endogenous MSCs originated from other organs are reported to participate in wound healing of the skin. In fact, early studies on adult stem cells in the dermal compartment showed that progenitors from bone marrow are chemokine-dependently recruited to the site of skin injury and participate in skin restoration. These cells express mesenchymal markers (collagen I, fibronectin) and are mainly understood as progenitors of fibroblasts; therefore, they are designated “fibrocytes” (Bucala et al. 1994). Fibrocytes constitutively produce ECM components as well as ECM-modifying enzymes and can further differentiate into myofibroblasts playing a crucial role in wound healing (Abe et al. 2001; Quan et al. 2004). Another study showed that upon wounding, stimulated bone-marrow-derived stem cells traffic through both wounded and non-wounded skin and incorporate into skin compartments (Badiavas et al. 2003). This concept was strongly supported by a study of Opalenik and Davidson (2005). Using a luciferase and b-galactosidase reporter under the control of collagen I a2 chain, the authors were able to show that the transplanted donor bone-marrow-derived cells occurred in the granulation tissue of recipient mice, differentiated to fibroblasts, and contributed significantly to total collagen I production during later stages of repair (Opalenik and Davidson 2005). Very recently, with a similar experimental strategy, these bone-marrow-derived cells have convincingly been proven to be bone-marrow-derived endogenous MSCs (Seppanen et al. 2013). Notably, ceramide-1-phosphate (C1P) which is upregulated during tissue damage was identified as an important chemoattractant for the recruitment of bone-marrow-derived MSCs to the site of injury (Kim et al. 2013). All these studies suggest that endogenous MSCs are native constituents of the wound bed and play a crucial role in cutaneous wound healing.
14.2.2 Therapeutic Function and Cellular Mechanisms of Transplanted MSCs in Wound-Healing Repair and Skin Aging
Despite the lack of knowledge on physiological functions of dermal MSCs in the skin in situ, the therapeutic benefits of transplanted MSCs in accelerating wound healing have been extensively documented in humans and various animal models regardless of the source of MSCs (skin, bone marrow, adipose tissue, skeletal muscle, umbilical cord blood, gingival, dental pulp, Wharton’s jelly, synovial, amniotic fluid) and donor (autograft, allograft, xenograft) (De Bari et al. 2001; Falanga 2012; Falanga et al. 2007; Heo et al. 2011; In’t Anker et al. 2003; Jiang et al. 2013; Miura et al. 2005; Qi et al. 2014; Sasaki et al. 2008; Stoff et al. 2009; Tark et al. 2010; Wan et al. 2013; Williams et al. 1999; Wu et al. 2007; Xie et al. 2013; Yew et al. 2011; Zhang et al. 2010; Ksander et al. 2014). MSCs apparently also enhance the quality of adult wound healing with reduced scar formation and regeneration of skin appendages (Qi et al. 2014; Stoff et al. 2009; Wu et al. 2014; Jackson et al. 2012; Liu et al. 2014).
By the release of a variety of bioactive trophic molecules and immunosuppressive functions, MSCs distinctly contribute to establish a regenerative microenvironment, which stimulates the proliferation of lineage-specific cells such as fibroblasts, epidermal cells, and endothelial cells to repair and restore damaged tissue. The MSC-imprinted regenerative microenvironment also stimulates and stabilizes angiogenesis and vessel formation and dampens the unrestrained activation of proinflammatory M1 macrophages eventually leading to scar-reduced or even scarless healing in adults. Here we summarize the major mechanisms that MSCs participate in improving wound healing (Fig. 14.2).
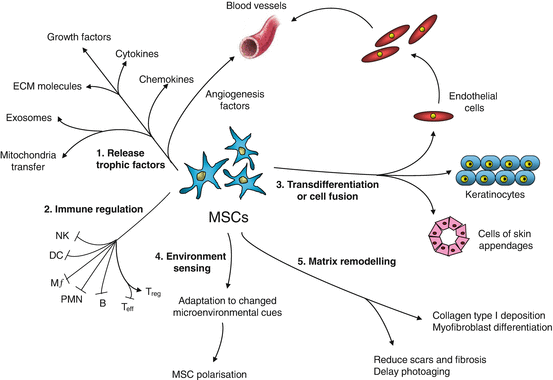
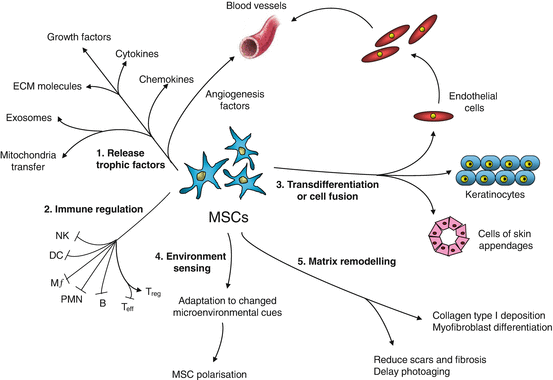
Fig. 14.2
Mechanisms of MSCs accelerated wound healing. MSCs participate in wound repair via several beneficial mechanisms, including (1) release of trophic factors, (2) suppression of overwhelming inflammation, (3) transdifferentiation into or fusion with specialized cells, (4) sensing of the microenvironment, and (5) remodeling extracellular matrices. ECM extracellular matrix, Mf macrophages, PMN polymorphonuclear neutrophil, DC dendritic cells, NK natural killer cells, Teff effector T cells, Treg regulator T cells
14.2.3 Paracrine Trophic Effects
Topical application and local or intravenous injection of MSCs for the treatment of acute and chronic wounds result in accelerated wound closure with increased epithelialization, granulation tissue formation, and angiogenesis (Jiang et al. 2013; Falanga et al. 2007; Heo et al. 2011; Wu et al. 2007). Although there is evidence for MSC differentiation in the wound, most of the therapeutic effects are likely due to MSCs releasing soluble factors that regulate local cellular responses to cutaneous injury (Hocking and Gibran 2010). MSCs are described as a factory of cytokines, chemokines, and ECM molecules (Caplan and Dennis 2006) or even a “drugstore” during injury (Caplan and Correa 2011; Murphy et al. 2013).
Chen et al. (2008) found that compared to dermal fibroblasts, BM-MSCs released vascular endothelial growth factor (VEGF), insulin-like growth factor-1 (IGF-1), epidermal growth factor (EGF), keratinocyte growth factor (KGF), angiopoietin-1, stromal cell-derived factor-1 (SDF-1), macrophage inflammatory protein-1 (MCP-1), and erythropoietin, which are essential for physiological wound healing. Moreover, in contrast to noneffective fibroblast-conditioned medium, BM-MSC-conditioned medium significantly enhanced migration and proliferation of macrophages, keratinocytes, and endothelial cells in vitro and in vivo and enhanced wound healing in a mouse model of excisional wound (Chen et al. 2008). Injected MSCs accelerate wound healing via the production and release of transforming growth factor beta (TGF-β and VEGF which induce myofibroblast differentiation and angiogenesis (Jiang et al. 2013; Nemeth et al. 2010; Popova et al. 2010; Salvolini et al. 2010a). Coculture experiments with dermal MSCs and endothelia cells suggest that MSCs have the potential to activate endothelial cells. This activation is mediated via the release of VEGF and NO with subsequent alteration of the endothelial barrier overall facilitating the transmigration of MSCs across the endothelium to the wound site (Salvolini et al. 2010b). Injection of TNF-α-activated human AT-MSCs accelerate wound closure, angiogenesis, proliferation, and infiltration of immune cells into the cutaneous wound through paracrine mechanisms involving IL-6 and IL-8 in a rat excisional wound model (Heo et al. 2011). Conditioned medium from AT-MSCs stimulates collagen synthesis and promotes proliferation and migration of human keratinocytes and fibroblasts during the wound-healing process (Lee et al. 2012; Rose et al. 2008). Interestingly, the synthesis of therapeutically relevant trophic factors such as IL-6, SDF-1, HGF, and VEGF was induced in MSCs following activation of the toll-like receptor 3 (TLR3) (Mastri et al. 2012). The ligand of TLR3 polyinosinic:polycytidylic acid (poly I:C), in fact, stimulates wound healing (Lin et al. 2012). These data in conjunction with the assumption that danger signals like virus-derived double-strand RNA (a TLR3 ligand) of virally induced tissue damage occur in wounds would support the notion that TRL3 activation on MSCs enhances tissue repair.
Dermal fibroblasts and myofibroblasts are mainly responsible for wound contraction, extracellular matrix deposition, and scar tissue remodeling during tissue repair (Smith et al. 2010). In response to cocultured MSCs, dermal fibroblasts upregulate integrin a7 and downregulate ICAM1, VCAM1, and MMP11 expression suggesting that MSCs may provide important early signals to enhance dermal fibroblast responses to enhance tissue repair after cutaneous injuries (Smith et al. 2010). In this context, MSCs enhance the directed migration of dermal fibroblasts to a gradient of soluble chemotactic agents released by MSCs (Rodriguez-Menocal et al. 2012). Notably, the migration of fibroblasts was found to be dramatically reduced in “wound” scratch assays on cocultures of unperturbed MSCs with fibroblasts isolated from tissue of chronic wound patients and lowest when both MSCs and fibroblasts stem from biopsies of chronic wound patients (Smith et al. 2010). These data suggest that both fibroblasts and MSCs isolated from chronic wounds – most likely due to the hostile microenvironment of chronic wounds (Wlaschek and Scharffetter-Kochanek 2005) – reveal distinctly impaired functions critical for proper tissue repair. In addition to the paracrine-mediated enhanced fibroblast migration, MSCs from human exfoliated deciduous teeth were found to increase proliferation and collagen synthesis of dermal fibroblasts (Ueda and Nishino 2010).
Besides the conventional secretion of soluble proteins, MSC-derived exosomes have recently been identified to effectively serve as paracrine mediators in accelerating tissue repair and regeneration. This conclusion was based on the interesting observation that the active components consisting of 50–200 nm-sized complexes which were isolated from conditioned medium of human embryonic stem cells derived from MSCs or fetal MSCs have the potential to markedly reduce the infarct size in a mouse model of myocardial ischemia/reperfusion (MI/R) injury, if intravenously infused 5 min before reperfusion (Lai et al. 2010a, b). The MSC-secreted complexes were identified as exosomes, organized as bi-lipid membrane vesicles of endosomal origin containing proteins and RNA. Exosomes are secreted through fusion of multivesicular bodies with MSC cell membrane. The exosomes’ cargo is efficiently protected from degradation by proteases and RNase and uptaken by recipient cells via endocytosis or membrane fusion (Lai et al. 2011).
Impaired mitochondrial function plays an essential role in aging, reduced tissue homeostasis, and repair. In 2006, it was reported that mitochondria and mtDNA can be actively transferred from human BM-MSCs to mammalian cells with nonfunctional mitochondria without cell fusion, and this transfer, in fact, rescues the aerobic respiration (Spees et al. 2006). This was confirmed in vitro by two subsequent studies showing that mitochondria were transferred from human BM-MSCs to cocultured rat cardiomyocytes (Plotnikov et al. 2008) or human osteosarcoma cells (Cho et al. 2012) without functional mitochondria. The evidence that the transfer of intact mitochondria can contribute to tissue repair in vivo was provided very recently. Islam and colleagues (2012) reported that human and mouse BM-MSCs attached to LPS-injured mouse alveolar epithelial cells transfer intact mitochondria by forming connexin-43-containing gap junctions, nanotubes (e.g., filopodia), and microvesicles (e.g., exosomes) and, thus, contribute to the repair of an injured lung in vivo (Islam et al. 2012; Prockop 2012). Whether the transfer of mitochondria from MSCs to injured or senescent cells occurs during wound healing of the skin is currently unclear and needs further investigation.
14.2.4 Angiogenesis
Angiogenesis is an essential process in tissue repair, regeneration, and reconstruction. Signals that control endothelial cell proliferation and differentiation have been studied on MSCs themselves or on their target tissues and organs. MSC differentiation into endothelial cells can be practically accomplished by exposure to angiogenic factors in vitro (Oswald et al. 2004). Exposure to VEGF evokes the differentiation of MSCs into endothelial-like cells, expressing VEGFR-1, VEGFR-2, and von Willebrand factor (vWF) as well as the formation of the characteristic capillary-like structures (Oswald et al. 2004). Forced expression of secreted Frizzled-related protein-1 enhanced MSCs surrounding neovessel in vivo and strongly increased PDGF-BB expression in MSCs and enhanced b-catenin-dependent cell-cell contacts between MSCs themselves and epithelial cells or smooth muscle cells in vitro (Dufourcq et al. 2008). After injury, the level of TGF-β1 in the wound dramatically increases and plays a pivotal role in the regulation of angiogenesis. TGF-β1 stimulated the synthesis of VEGF and the phosphorylation of Akt and ERK1/2 in MSCs (Wang et al. 2008). On the other hand, the paracrine factors, including TGF-β1, VEGF, bFGF, angiogenin, procathepsin B, IL-11, and BMP2, all secreted by MSCs, affect endothelial cell migration, ECM invasion, proliferation, and survival in vitro (Potapova et al. 2007) and induce angiogenesis and enhance wound healing in vivo (Wu et al. 2007).
Recently, MSCs have been proposed to arise from perivascular stromal cells including pericytes, which are localized on the abluminal side of blood vessels, immediately opposed to endothelial cells (Crisan et al. 2008; Traktuev et al. 2008). BM-MSCs express pericyte markers such as α-SMA, desmin, calponin, smoothelin, and NG2 (Au et al. 2008) and can home to skin wound sites where they may exert pericyte functions to support and stabilize new vessel formation (Ozerdem et al. 2005). Au et al. (2008) found that BM-MSCs elongated and co-aligned in close proximity to vascular tubelike structures. MSC migration towards vascular cells depends on PDGF-BB, a growth factor that also enhances pericyte migration. In vivo data suggest that pericytes are attracted to newly formed vessels (Au et al. 2008). The preferred perivascular localization of MSCs may be related to their origin and interaction with vascular structures after homing. Pericyte coverage is important for the stability and remodeling of blood vessels in wound healing (Bergers and Song 2005).
14.2.5 Immune Regulation in the Inflammatory Phase of Tissue Repair
MSCs are low immunogenic cells. Despite constitutive expression of MHC-I and upregulation of MHC-II induced by IFN-γ, human MSCs fail to stimulate proliferation of allogeneic lymphocytes in vitro, even in the presence of CD28-stimulating antibodies or B7 costimulator (Prockop et al. 2010), which makes allogeneic transplantation of MSCs possible. MSCs have shown promising regulatory effects on both adaptive and innate immune systems in general and during tissue repair in particular. MSCs are reported to suppress proliferation and function of T-effector cells and induce their apoptosis by indoleamine 2,3-dioxygenase (IDO)-mediated tryptophan depletion (Meisel et al. 2004; Selmani et al. 2008; Akiyama et al. 2012; Ren et al. 2008); promote the generation of regulatory T cells (Selmani et al. 2008; Kavanagh and Mahon 2011; Sun et al. 2011; Ghannam et al. 2010); reduce B-cell activation and proliferation (Corcione et al. 2006; Maby-El Hajjami et al. 2009); suppress differentiation, maturation, and antigen-presenting functions of dendritic cells (Nauta et al. 2006; Aggarwal and Pittenger 2005); inhibit proliferation and cytotoxicity of natural killer cells (Selmani et al. 2008; Sotiropoulou et al. 2006; Aggarwal and Pittenger 2005); induce macrophage polarization from a proinflammatory M1 to an anti-inflammatory M2 state (Dayan et al. 2011; Jiang et al. 2013; Kim and Hematti 2009; Qi et al. 2014; Zhang et al. 2010); and inhibit neutrophil apoptosis and activation (Jiang and Scharffetter-Kochanek unpublished data) (Raffaghello et al. 2008; Tso et al. 2010). Several recent reviews provide further information (Prockop et al. 2010; Nasef et al. 2008; Atoui and Chiu 2012; Bunnell et al. 2010; Shi et al. 2010).
TNF-α released from proinflammatory M1 macrophages stimulates inducible nitric oxide synthase (iNOS) with subsequent generation of microbicidal NO• to combat invading microbes during early phases of wound healing (Daley et al. 2010). By contrast to scarless fetal wound healing with little or no TNF-α release, TNF-α concentrations in acute physiological healing result in enhanced microbicidal activity at the expense of enhanced scar formation. Also persisting overexpression of TNF-α as observed in chronic wounds results in the nonhealing state (Sindrilaru et al. 2011). A vicious cycle of persistent high concentrations of reactive oxygen species and matrix metalloproteinase-1 activation with subsequent tissue breakdown and premature senescence of resident wound fibroblasts are responsible for the delay in tissue restoration (Sindrilaru et al. 2011). In addition, high TNF-α concentrations – via an autocrine loop – maintain and amplify proinflammatory M1 macrophage activation (Sindrilaru et al. 2011; Wang et al. 2006). MSCs significantly suppressed TNF-α release from macrophages in vitro and in vivo and thus contribute to the accelerated wound healing in various animal models (Pietila et al. 2012; Qi et al. 2014; Zhang et al. 2010).
TNF-α-stimulated gene 6 protein (TSG-6) has recently been identified as a key anti-inflammatory molecule released from activated MSCs. TSG-6 released from intravenously infused human MSCs decreased inflammatory responses, reduced infarct size, and improved cardiac function in experimental myocardial infarction (Lee et al. 2009). Moreover, through the interaction with CD44 on macrophages, MSC-derived TSG-6 attenuates zymosan-induced mouse peritonitis due to its ability to decrease the TLR2-mediated nuclear translocation of NFκB in resident macrophages (Choi et al. 2011). Recently, our group has demonstrated that TSG-6 released from MSCs improves wound healing by limiting macrophage activation, inflammation, and scar formation in a murine model of full-thickness skin wounds (Qi et al. 2014).
14.2.6 Transdifferentiation
Stem cells are believed to have tissue regeneration capacity during injury through self-renewal, engrafting, differentiation, and transdifferentiation into histogenetically distinct tissue cells. In vitro, MSCs have shown great potential to transdifferentiate into non-mesenchymal lineages such as endothelial cells (Oswald et al. 2004), keratinocytes (Fu et al. 2006; Sasaki et al. 2008), hepatocytes (Stock et al. 2010; Wu and Tao 2012; Liang et al. 2012), cardiomyocytes (Toma et al. 2002; Xu et al. 2004), and neurons (Joannides et al. 2004; Yang et al. 2004). However, the transdifferentiation capacity of MSCs in vivo is still under debate. A mesenchymal population resident in neonatal murine dermis has the potential for transdifferentiation into epidermal cells in a three-dimensional culture (Crigler et al. 2007). Approximately 10 % of ex vivo-expanded murine BM-MSCs differentiate into CD31+ and VEGF+ endothelial cells in vivo (Al-Khaldi et al. 2003). Fu and colleagues (2006) have demonstrated the differentiation of MSCs into vascular endothelial cells, epidermal cells, or sweat gland cells under different lineage-specific culture conditions. More importantly, a few percent of autograft MSCs transdifferentiated into vascular endothelial cells in granulation tissues, sebaceous duct cells, and epidermal cells in regenerated skin in vivo in a full-thickness wound model in minipigs (Fu et al. 2006; Li et al. 2006). These authors also showed that wound-healing quality, such as the speed of reepithelialization, the number of epidermal rete ridges, thickness of the regenerated epidermis, the morphology, the number, and the arrangement of microvasculature, fibroblasts, and collagen, were markedly enhanced (Fu et al. 2006). These authors even managed to regenerate functional sweat gland-like structures based on transplantation of ex vivo predifferentiated human BM-MSCs (Sheng et al. 2009). In addition, Sasaki et al. (2008) demonstrated that MSCs were recruited into wounded skin and contribute to wound repair by transdifferentiation into multiple skin cell type in vivo. This conclusion was based on the observation that markers for keratinocytes, endothelial cells, and pericytes were detected on donor GFP-tagged MSCs (Sasaki et al. 2008). However, whether these cells retained their mesenchymal markers and whether they were functional keratinocytes or endothelial cells was not addressed in this study.
Unfortunately, clinical observations so far have failed to demonstrate a therapeutically relevant level of long-term engraftment or transdifferentiation of transplanted MSCs. The concept of MSC transdifferentiation is still under discussion and may not reflect what is occurring during tissue repair in vivo (Sanges et al. 2011; Zech 2005; Lee et al. 2009). For example, BM-MSCs express cardiac-specific markers, retain the stromal phenotype, and do not become functional cardiomyocytes, which generate action potentials or display ionic currents (Rose et al. 2008). Schneider and colleagues (2008) showed that multipotent human MSCs failed to transdifferentiate into E-cadherin- or cytokeratin-expressing cells under optimized organotypic culture conditions for keratinocytes but differentiated into myofibroblast-like cells with the key ability to contract and organize extracellular matrices (Schneider et al. 2008). Despite the viability and evident three-dimensional epidermis-like growth pattern, MSCs showed a persistent expression of mesenchymal, but not of epithelial markers, thus indicating a lack of epidermal differentiation (Schneider et al. 2008). Therefore, the authors argued that MSCs might rather contribute to wound-healing processes like wound contraction and reorganization of the newly deposited extracellular matrix, but do not significantly contribute to transdifferentiation into keratinocytes. Nevertheless, the partial cellular reprogramming, leading to the acquisition of at least some characteristics of the desired lineage, may possibly contribute to the tissue regeneration capacity of MSCs (Rose et al. 2008). In fact, emerging studies have suggested that the observed tissue regeneration of transplanted MSCs could be due to cell fusion of MSCs with resident cells at the wound site, thus enhancing cellular plasticity with subsequent changes in their developmental potential (Sanges et al. 2011; Zech 2005). It will be interesting for the future studies to investigate whether MSCs simply fuse with histologically distinct resident skin cells during wound healing.
14.2.7 Microenvironment Sensing
MSCs are evolutionary optimized to sense the microenvironment to protect the supportive tissue-specific niche for other stem cells and themselves (Meraviglia et al. 2014; Murphy et al. 2013). In simple organisms such as planarians, hydra, and starfish, stem cells in the mesenchyme (the blastema) are well positioned to interpret cues from the environment and to execute decisions about the direction of wound repair and tissue regeneration (reviewed in (Stappenbeck and Miyoshi 2009)). Wnt, BMP, and Hox signals, neuropeptides, and microRNAs are suggested to be important regulators in sensing the anterior-posterior axis. However, how mammalian MSCs communicate with the overlying epithelium and inflammatory cells in the mesenchyme during wound healing is not well understood.
Emerging studies suggest that pattern recognition receptors (PRRs) including pathogen-associated molecular patterns (PAMPs) and damage-associated molecular patterns (DAMPs), for example, toll-like receptors (TLRs), may qualify as environmental sensor of MSCs (Hwa Cho et al. 2006; Pevsner-Fischer et al. 2007; Raicevic et al. 2010; Mastri et al. 2012; Nurmenniemi et al. 2010), although their expression level on MSCs is very low. A recent report demonstrated that TLR4-primed MSCs were proinflammatory (MSC1), while TLR3-primed MSCs were immunosuppressive (MSC2) (Waterman et al. 2010). The authors suggested a new MSC1-MSC2 polarization paradigm, which has attracted much attention in the field (Keating 2012; Bunnell et al. 2010). However, in apparent contradiction to the findings that TLR4-primed MSCs are proinflammatory by Waterman et al. (2010), several studies have reported beneficial effects of MSC treatment in animal models of sepsis or LPS-induced lung injury, in which MSCs were administered within 1 h following LPS challenge (Nemeth et al. 2009; Nemeth et al. 2010; Delarosa et al. 2012; Gonzalez-Rey et al. 2009). Based on the therapeutic benefit observed in these experimental models, the conclusion was drawn that high concentrations of LPS did not polarize MSCs towards a proinflammatory phenotype. In addition to these in vivo findings, upon the addition of IFN-γ and LPS (TLR4 ligand) to MSC-macrophage cocultures, MSCs exhibited an excellent suppressive effect on TNF-α release from cocultured macrophages (Jiang et al. 2013; Qi et al. 2014). It is possible that the dosage and duration of TLR ligands that MSCs are exposed to and model inherent differences are critical for MSC polarization. This also suggests that other mechanisms exist to fine-tune the environment-sensing capability of MSCs. Overall the concept of a sensing mechanism in MSCs is clinically relevant as this would imply that adaptive responses will be provided, eventually even qualifying MSCs as versatile “adaptive drugstores,” which – in contrast to the application of recombinant growth factors or a designed cocktail thereof – would have the advantage to perfectly adapt to the specific needs of defined wounds.
14.2.8 Matrix Remodeling
MSCs also showed promising effects on the synthesis, deposition, and remodeling of the extracellular matrix reducing scar tissue formation and preventing skin aging. BM-MSCs were shown to attenuate bleomycin-induced skin (Wu et al. 2014) and lung fibrosis (Ortiz et al. 2003) in mice. The detailed mechanisms of how MSCs reduce scar formation during the remodeling phase of wound healing are far from being fully defined. It is most likely that besides dampening inflammation in early phases of wound repair, antifibrotic factors released by MSCs contribute to further reduce fibrogenic responses (Jackson et al. 2012). Recently, we and others reported that human bone-marrow-derived MSCs injected at the wound site released the anti-inflammatory factor TSG-6 and concomitantly modulate the TGF-β3 to TGF-β1 ratio, resulting in reduced scar formation in murine full-thickness wounds (Liu et al. 2014; Qi et al. 2014).
Moreover, MSCs exert beneficial effects for the prevention and reduction of skin aging. Transplantation of dermal MSCs from young BALB/c mice into aged mice resulted in a substantial increase in dermal thickness and collagen type I content in the dermis (Xue and Li 2011). Subcutaneous injection of AT-MSCs significantly stimulated collagen synthesis and angiogenesis and also increased dermal thickness, collagen density, and fibroblast numbers in the skin of hairless mice (Heo et al. 2011). These studies suggest that MSCs, especially dermal MSCs, play an important role in improving the phenotype of aged skin, which – if untreated – is characterized by severe atrophy, reduced collagen deposition, and rarefaction of elastic fibers with reduced skin resilience and enhanced wrinkle formation (Kligman and Kligman 1986; Fisher et al. 2008). In vitro data suggest that by the activation of TGF-β/Smad and p38 MAPK signaling pathways, dermal MSCs secrete various growth factors that control and reroute senescent neighboring fibroblasts to secrete and synthesize collagen or elastin, enhance extracellular matrix deposition, and thus may contribute to improve wrinkles and strengthen overall skin elasticity (Wang et al. 2008). Conditioned medium from AT-MSCs stimulated both collagen synthesis and migration of dermal fibroblasts, which improved the wrinkling and accelerated wound healing in animal models. MSC-conditioned medium also protected dermal fibroblasts from oxidative stress induced by chemicals and UVB irradiation and even has the capacity to reverse the noxious UVB-induced suppression of fibroblast proliferation, reduced collagen type I synthesis, and enhanced proteolytic MMP-1 activity in dermal fibroblasts towards tissue homeostasis and rejuvenation (Kim and Hematti 2009).
A contradiction is currently obvious for effects of MSCs on myofibroblasts differentiation and collagen deposition. In wound healing and photoaging studies, MSCs were found to induce myofibroblasts differentiation, collagen deposition, and granulation tissue formation and enhance dermal thickness (Xue and Li 2011; Jiang et al. 2013; Wan et al. 2013), while in studies on fibrosis and scar formation, MSCs seem to have the opposite effects with reduction in collagen synthesis and reduced myofibroblast differentiation (Liu et al. 2014; Qi et al. 2014; Wu et al. 2014). It is likely that microenvironmental differences of the studied models may be responsible for the apparently opposite MSC responses, and it will be especially exciting to decipher the underlying mechanisms in future studies.
In summary, MSCs exert their effects by multiple ways that stimulate tissue recovery on many important levels, including stimulation of endogenous progenitor cells, suppression of apoptosis of vulnerable cells, remodeling of extracellular matrices, and stimulation of new blood vessel formation. However, the majority of these mentioned studies were performed in vitro, and often the MSC numbers assessed in culture were higher when compared to physiological or therapeutic situations in vivo. Therefore, the identification of MSC-specific markers with subsequent development of transgenic models to trace endogenous MSCs or the refinement of state-of-the-art technology to track exogenously added MSCs in skin and tissues is urgently required for meaningful in vivo studies to clearly dissect MSC functions in situ in physiological and pathologic conditions of tissue repair and aging.
14.3 Impact of Mesenchymal Stem Cell Aging on Wound Healing
The cellular mechanisms of aging of skin stem cells (Chap. 8) and in particular MSCs (Chap. 11) have been detailed in the previous chapters. Here we would like to emphasize the consequence of MSC aging on impaired wound healing. The most obvious and striking evidence that aging plays an important role in wound healing comes from the fact that fetal wounds can heal rapidly without scars, while in adults wound healing decreases with aging and frequently though not always reveal enhanced fibrosis and scar formation. Among other reasons, this most likely reflects changes in the functional state of mesenchymal stem cells.
14.3.1 Age-Induced Changes in MSCs and Impact on Wound Healing
Like other stem cells, MSCs have evolutionary developed sophisticated mechanisms to protect themselves during aging. For example, MSCs have a high resistance to oxidative-stress-induced death, which correlates with the low level of intracellular reactive species by effective ROS scavenging, constitutive expression of antioxidant enzymes required to counteract oxidative stress, and high levels of total intracellular glutathione (Valle-Prieto and Conget 2010). Human MSCs also constitutively express methionine sulfoxide reductase A at high levels, an enzyme which is crucial for the repair of oxidized proteins and for the recovery of methionine residues that efficiently serve as scavengers for oxidants (Salmon et al. 2009). However, a recent study has shown that excessive activation of Wnt/b-catenin signaling induces MSC aging through promoting the intracellular production of ROS despite higher expression of antioxidant enzymes (Zhang et al. 2013). This suggests that the aging process inevitably brings detrimental changes to MSCs. Aging affects MSC proliferation and differentiation and their cell surface marker expression profile. The declined capacity for wound repair with age may be due to age-related changes in MSCs (Fig. 14.3).
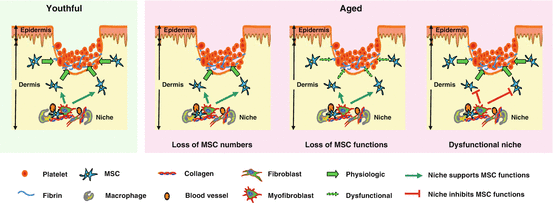
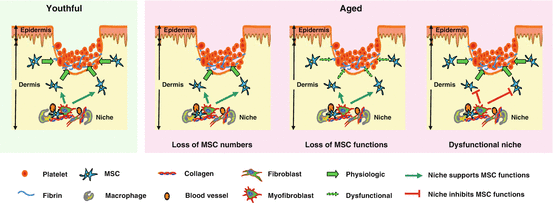
Fig. 14.3
Impact of aging on MSCs. The decline of MSC numbers and functions in wound repair with age can be assigned to age-related changes at several levels, including (1) decreased numbers of MSCs, (2) decline in MSC functions required for wound healing, and (3) persisting proinflammatory oxidative niche/microenvironment
14.3.2 Decrease of MSC Numbers Across the Life Span
Like other adult stem cells, MSCs are a rare population among other cells in their resident tissues. For example, clonal analysis revealed that 6.4 % of the single-cell-derived dermal clones were tripotent and that dermal stem cells represent approximately only 0.3 % of human dermal foreskin fibroblasts (Chen et al. 2007). Unfortunately, with such a small pool, the number of MSCs declines dramatically with age. MSCs in human bone marrow diminish in number almost exponentially from birth (1 in 10,000 nucleated cells) to the eighth decade of life (1 in 2,000,000 nucleated cells) (Haybesworth et al. 1994). With increasing age, osteoprogenitor cells derived from MSCs in rat bone marrow display decreased potential for colony formation and size (Dodson et al. 1996) and a reduced ability to form bone (Quarto et al. 1995). The same conclusion was obtained in human MSCs. By comparing BM-MSCs from old and young human donors, Stenderup and colleagues (2003) found aging was associated with decreased proliferative capacity of osteoprogenitor cells, thus the decreased osteoblastic cell number (Stenderup et al. 2003). In the skin compartment, it has recently been reported that the abundance and differentiation potential of human skin-derived precursors (SKPs), a subpopulation of mesenchymal stem cells with the capacity to differentiate into neurons and smooth muscle cells, decrease sharply with age (Gago et al. 2009). The authors found that SKPs are extremely difficult to isolate, expand, and differentiate when obtained from the elderly (Gago et al. 2009). However, it is still unclear whether human fetal skin differs in MSC numbers, location, and function from adult skin and if so which mechanisms is responsible for such sharp decline in MSC numbers in adult skin of the elderly. Fetal tissue has the ability to rapidly heal skin wounds without a scar unlike adult tissue. Differences in MSCs between fetal and adult skin in the context of wound healing has not been extensively studied, but will be an important area for further investigation.
14.3.3 Loss of Function in Aged MSCs
Experts in the field argue that impaired wound healing in aged skin is rather induced by impaired stem cell mobilization or reduced ability to respond to proliferative signals than reduced stem cell numbers per se (Sharpless and DePinho 2007; Zouboulis et al. 2008), a situation similar to hematopoietic stem cells (Geiger et al. 2013) and skeletal muscle satellite cells in aged individuals (Rando 2006).
It is well known that aging perturbs cytokine and hormone networks. Similarly, aged MSCs reveal a significantly altered cytokines prolife that is less effective in tissue repair (Galderisi et al. 2009; Kretlow et al. 2008; Crisostomo et al. 2006). By contrast to young BM-MSCs, aged BM-MSCs with significantly higher p16INK4a expression downregulate the secretion of IGF-1, fibroblast growth factor (FGF-2), VEGF, and hepatocyte growth factor (HGF), leading to a decline in their capacity to induce angiogenesis exemplified by reduced tube-forming on Matrigel (Sen et al. 2002). Choudhery et al. (2012) also showed that compared to young MSCs, aged MSCs display significantly reduced SDF-1 and VEGF expression (Choudhery et al. 2012) with severe impact on wound repair. SDF-1 is involved in homing, engraftment, neovascularization, and cell proliferation. VEGF increases vascular permeability, promotes angiogenesis, attracts monocytes to the site of injury, and is also involved in neovascularization (Matsumoto et al. 2005; Tang et al. 2005).
Furthermore, there is an age-related decline in overall MSC “fitness” as suggested by Stolzing et al. (2008). They found human BM-MSCs from older donors to have reduced CFU-F capacity and increased oxidative damage, ROS levels, and p21 and p53 expression (Stolzing et al. 2008). In line with this, BM-MSCs from aged mice revealed reduced angiogenesis, proliferation, and antiapoptotic effects with overall reduced wound healing promoting potential (Choudhery et al. 2012). The authors compared BM-MSCs from young and aged C57BL/6 mice. By contrast to aged MSCs, they found a significantly higher expression the antiaging NAD-dependent histone deacetylase Sirt1 in young MSCs. Aged MSCs revealed a reduction in VEGF, SDF-1, and AKT and a consistently increased expression of p53, p21, Bax, and p16INK4a. Tube formation, wound healing, and proliferative potential of MSCs from young mice were significantly better compared to that of MSCs from aged mice. This study suggests that the age-related increase in the expression of apoptotic and senescent genes, with the concomitantly occurring decrease in Sirt1 expression, is at least to some extent responsible for impaired MSC functions. In mammalian cells, Sirt1 is known to enhance proliferation by the suppression of p53 (Chua and Laurent 2006) and to inhibit cell apoptosis and senescence (Jung-Hynes and Ahmad 2009). Lamin A has been identified as a direct activator of Sirt1 (Liu et al. 2012) and if mutated leads to premature aging as earlier reported for Hutchinson-Gilford progeria syndrome (Liu et al. 2011). This suggests that lamin A via Sirt1 induction possibly contributes to the regenerative ability of MSCs and – if disrupted – may play a critical role in MSC aging. In line with these findings, Park et al. (2005) reported that the adipogenic differentiation potential of human MSCs sharply declines at the end of their proliferative life span. In senescent MSCs, the basal phosphorylation level of ERK was also significantly increased, a finding commonly shared by senescent cells (Park et al. 2005). A striking observation suggests that aged bone-marrow cells rather inhibit than induce skin wound vascularization. Accordingly, lineage-negative (lin−) bone-marrow cells from young mice greatly increase vascularization and decrease wound size in healing-impaired mice. In contrast, as with lin− cells from obese diabetic mice, lin− cells from old mice had no significant effect on wound size, but profoundly impaired neovascularization in wounds (Schatteman and Ma 2006). These studies suggest transplantation of aged MSCs is likely to result in reduced therapeutic effects in older patients and may actually be detrimental.
Recently, miRNAs were found to be involved in replicative senescence (Li et al. 2011; Hackl et al. 2010; Scheid et al. 2002) and physiological aging of MSCs (Li et al. 2011). The role of miRNAs in the proliferation and differentiation of MSCs in the context of wound healing has also been explored (reviewed (Guo et al. 2011)). Altered expression of a panel of miRNAs with upregulation of miR-31, −21, −223, −142, −205, −203, −18b, −19a, −130b, −16, −26b, −125b, and let-7f and downregulation of miR-133a, −181, −30a-3p, −193b, −30a-5p, −204, −200b, −96, −127, −181c, −182, and -130a was detected in injured tissue at the stage of active granulation formation in murine skin excision models (Zou et al. 2010; Shilo et al. 2007). Furthermore, Zou and colleagues (2010) found that TGF-β, a key growth factor elevated at the wound site, stimulates upregulation of miR-21 in MSCs, thus promoting proliferation and differentiation of MSCs in vitro. Consistently, knockdown of miR-21 in the wound bed delayed the healing process (Zou et al. 2010). These results suggest that miR-21 regulates gene expression and, subsequently, the behavior of MSCs in wound healing. It remains to be seen whether the changes of miRNA expression pattern during aging contribute to the decline in MSC potential and impaired wound healing and tissue regeneration in elderly.
14.3.4 Dysfunctional Microenvironment in Aged Skin
Skin homeostasis is gradually lost as a consequence of the intrinsic aging process, ultraviolet light, and other environmental threats resulting in a dramatic change in the structure of dermis. The most remarkable change is referred to the switch of dermal fibroblasts from a collagen-synthesizing phenotype to a more proteolytic phenotype (Varani et al. 2004), which clinically translates to the gradual appearance of wrinkles and severe skin sagging.
Senescent cells mainly dermal fibroblasts develop during intrinsic aging, as a result of a repetitive stress response or a combination thereof. Senescent fibroblasts are characterized by their resistance to apoptosis and the manifestation of their senescence-associated secretory phenotype (SASP) with the release of a vast variety of proinflammatory factors that alter the tissue microenvironment and may affect MSC functions (Campisi 2005). In addition, age-associated immunosenescence leads to an elevated endogenous inflammatory milieu (Gruver et al. 2007; Aw et al. 2007; Peters et al. 2009). We recently reported that the persistent inflammatory microenvironment in chronic venous leg ulcers with elevated TNF-α and unrestrained release of hydroxyl radical by macrophages results in the induction of senescent wound bed fibroblasts and impaired wound healing (Sindrilaru et al. 2011). It is tempting to speculate that such a hostile microenvironment also causes senescence and functional loss of MSCs, eventually promoting the state of never-healing chronic wounds.
BM-MSCs from aged donors displayed diminished long-term proliferation potential and elevated expression of vascular cell adhesion molecule-1 (VCAM-1; CD106) as a response to the inflammatory environment (Laschober et al. 2011). The authors demonstrated that moderate levels of inflammatory stimuli are interpreted by MSCs at a young age as instructive signals for osteoblastogenesis, whereas at old age, an inflammatory microenvironment may effectively suppress bone remodeling and repair by tissue-borne MSCs, while promoting adipogenic differentiation eventually leads to an increase in adipose tissue (Laschober et al. 2011).
Notably, reduced b1 integrin expression on MSCs as occurring during aging leads to reduced proliferation potential especially during wound healing (Piwko-Czuchra et al. 2009). Since b1 integrins play important roles in interacting with other resident cells and ECM molecules, it is most likely that changes in b1 integrin expression on aged MSCs disrupt downstream signaling and thus exert still not fully understood effects on MSC behavior during injury in the elderly.
Several lines of evidence suggest that defective neovascularization with aging is related to depressed signaling by hypoxia-inducible factor-1 (HIF-1), the master regulator of neovascularization, which regulates the expression of VEGF, SDF-1, and CXC chemokine receptor-4 (CXCR4). The function and mobilization of MSCs have been shown to decrease with decline in HIF-1 signaling with age, which may lead to depressed neovascularization and wound healing (Hoenig et al. 2008).
The aged MSCs themselves also have impact on other cell populations in the environment, for example, on fibroblasts. MSCs derived from healthy donors were significantly better than MSCs derived from chronic wound patients in stimulating directed fibroblast migration (Rodriguez-Menocal et al. 2012). Accordingly, the aging process is amplified by the vicious feedback loop between MSCs and their niche.
14.3.5 MSCs in Aging-Related Chronic Wounds: Chronic Wounds due to Intrinsic Aging
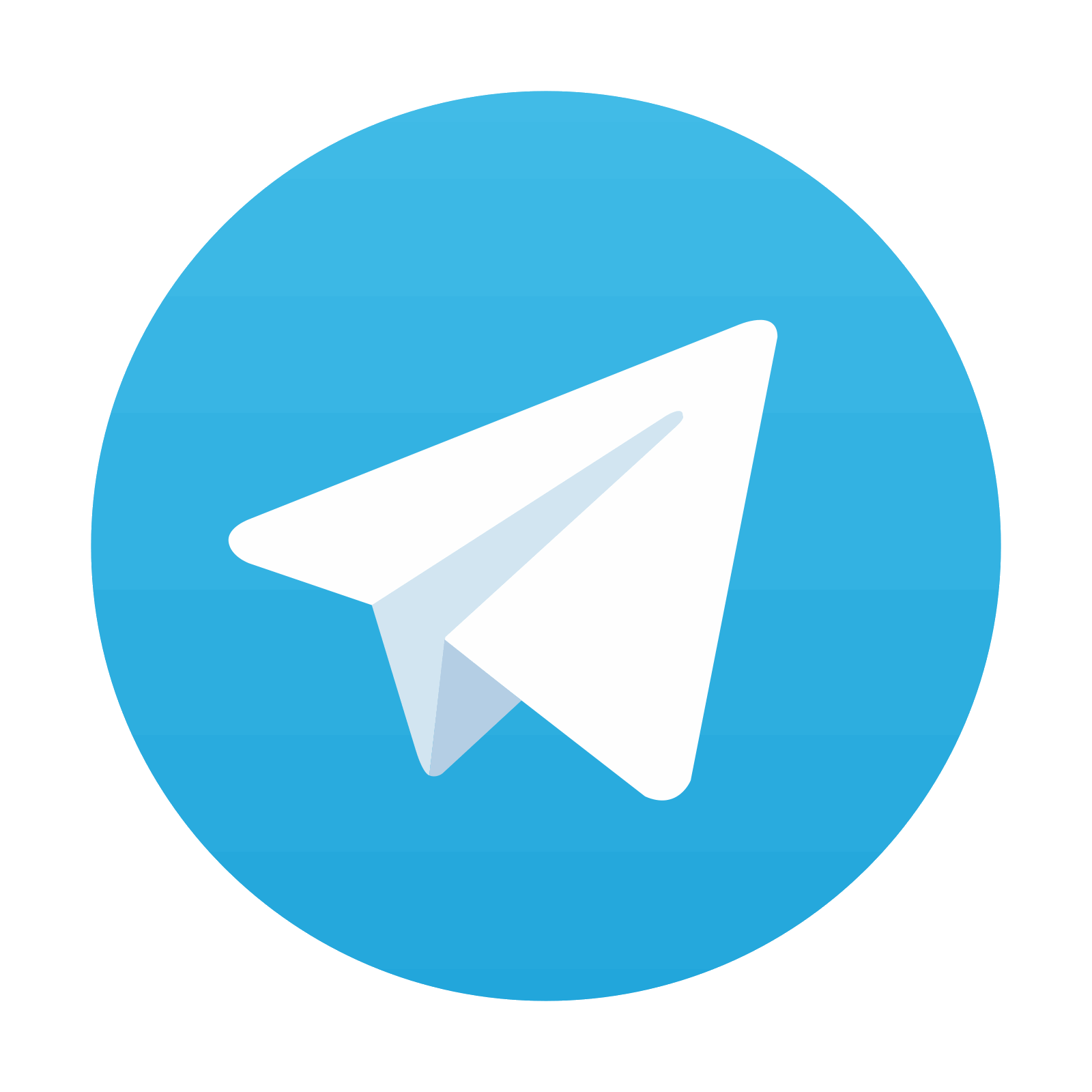
Stay updated, free articles. Join our Telegram channel
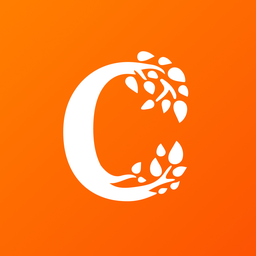
Full access? Get Clinical Tree
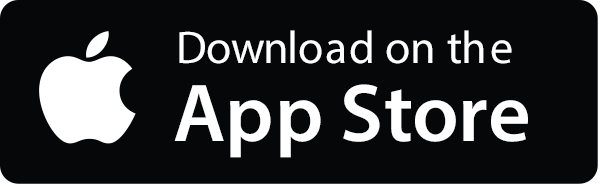
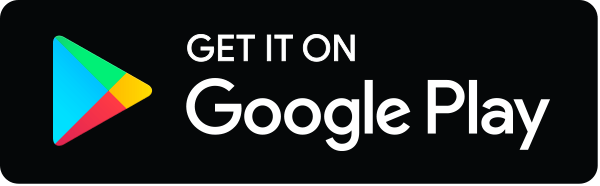