Fig. 10.1
Schematic representation of the surgical manipulations in (a) Roux-en-Y gastric bypass, (b) adjustable gastric banding and (c) vertical sleeve gastrectomy
The AGB technique involves the insertion of an adjustable silicone ring around the proximal aspect of the stomach, immediately below the gastro-oesophageal junction creating a small proximal pouch (Fig. 10.1). The volume of fluid in the band is adjusted through injections in a subcutaneous port and the procedure results in 20–25 % long-term weight loss [8, 9]. The VSG is fashioned through the reduction of gastric volume by the laparoscopic removal of 70–80 % of the stomach (Fig. 10.1). Previously, VSG was performed as part of the duodenal switch procedure, but is increasingly used as a stand-alone procedure that can cause a weight loss of 20–30 % in the long term [10]. Because of increased rates of postoperative and nutritional complications, the biliopancreatic diversion (BPD) and duodenal switch procedures are performed less frequently compared to the other procedures [8, 9].
The benefits of bariatric surgery extend beyond improvements in weight and glycaemic control (the latter are discussed later in this chapter); patients also exhibit reductions in overall and cardiovascular morbidity and mortality rates, as well as a reduction in cancer incidence in women [1, 2, 11]. Nevertheless, there are no data from RCTs to support the use of surgery for comorbidities that are frequently associated with obesity such as non-alcoholic fatty liver disease, subfertility, renal disease and functional impairment. In terms of obstructive sleep apnoea, AGB resulted in greater weight loss, but did not improve the apnoea–hypopnoea index significantly more than non-surgical weight loss therapies [12]. In the absence of sufficient evidence, the choice of technique depends on patient and multidisciplinary team preference, local expertise and funding.
Complications of Bariatric Surgery
As with any intervention, bariatric surgery is not without complications. The most serious complications associated with bariatric surgery include postoperative sepsis, anastomotic leaks, bleeding and venous thromboembolism, including fatal pulmonary embolism [13]. The risk of early mortality after bariatric surgery ranges from 0.1 to 2.0 % depending on the procedure [8]. The longitudinal assessment of bariatric surgery consortium reported a 30-day postoperative mortality rate of 0.3 % with RYGB [14]. Factors associated with increased mortality include male gender, age older than 65 years, reduced cardiorespiratory fitness levels, and limited surgeon experience [13].
Long-term nutritional deficiencies may occur in some bariatric surgery patients due to changes in the anatomy of the gastrointestinal tract with surgery [15]. Deficiencies in vitamin B12, folate and iron are not uncommon early after surgery and evidence of calcium, vitamin D and trace element deficiencies can also occur months to years after the procedure [15].
Overall, modern bariatric surgery has an acceptable risk/benefit profile, with careful patient selection and the availability of an appropriately experienced multidisciplinary team that is responsible for patient care in both the preoperative and postoperative periods.
Mechanisms Underlying Weight Loss
The first bariatric surgery techniques were developed during the 1950s. At the time surgeons attempted to design procedures that mechanically restricted the consumption of food and/or caused significant malabsorption of the consumed calories. Since then only RYGB, AGB and VSG have stood the test of time and are widely performed around the world. Intriguingly, these procedures appear to promote weight loss through mechanisms that are very different to those in the minds of the clinicians that originally designed them. These mechanisms will be described in this section and summarised in Table 10.1.
Table 10.1
Mechanisms of weight loss and glycaemic improvements after bariatric surgery
RYGB | VSG | AGB | |
---|---|---|---|
Appetite | ↓ | ↓ | ↓ |
Plasma ghrelin | ↑/↓/↔ | ↓ | ↑ |
Plasma GLP-1 | ↑ | ↑ | ↔ |
Plasma PYY | ↑ | ↑ | ↔ |
Plasma oxyntomodulin | ↑ | ? | ? |
Plasma CCK | ↔ | ↔/↑ | ? |
Plasma leptin | ↓ | ↓ | ↓ |
Gastric emptying | ↑/↓ | ↑ | ↔ |
Caloric malabsorption | Minimal for fat only | ? | ? |
Energy expenditure | ↑/↓/↔ | ↔ | ? |
Food preferences | ↓ Consumption of fat and sugar | ↓ Consumption of fat and sugar | ↔ or ↑consumption of fat and sugar |
Glycaemic improvements | Early and sustained, weight dependent and independent | Early and sustained, weight dependent and independent | Gradual and sustained, weight dependent |
Early postprandial insulin release | ↑, Early and sustained | ↑, Early and sustained | ↔ |
Insulin resistance | ↓ | ↓ | ↓ |
Plasma bile acids | ↑ | ↑ | ↔ |
Gut microbiota | Significant changes | ? | ? |
Appetite
Changes in appetite are reported within days following bariatric surgery. Increased satiety and decreased hunger postoperatively have been reported after the RYGB, VSG and LAGB [16–18]. This is very much in contrast with the reports of patients who consume similar amounts of calories through dieting and describe increases in hunger, decreases in satiation and preoccupation with food (e.g. [19, 20]). This observation suggests that bariatric surgery procedures have an advantageous effect on the control of food intake, in that through changes in physiology they assist the individual reduce their caloric intake and weight loss and maintain them in the long term.
Hypothalamic Signalling After Bariatric Surgery
Only one study so far has assessed the expression of key signalling elements within the hypothalamic nuclei of rodent models of bariatric surgery (e.g. pro-opiomelanocortin, neuropeptide Y, agouti-related peptide). The expression of orexigenic agouti-related peptide remained unchanged in rats undergoing VSG compared with sham surgery, whereas it increased in rats pair-fed to the VSG group [21]; this finding might suggest that the calorically restricted rats were hungry and the VSG were not. Gene expression studies have not been performed after RYGB or AGB. However, RYGB is effective even in patients with heterozygous mutations of the melanocortin 4 receptor gene (e.g. [22]). Therefore based on the currently available data, there is insufficient evidence in support of the hypothesis that bariatric surgery alters signalling within the hypothalamic nuclei controlling food intake.
Hormonal Signalling After Bariatric Surgery
There is substantial evidence that bariatric surgery reduces appetite through alterations in the gut–brain axis signalling. Following RYGB, the postprandial levels of the anorexigenic gut hormones glucagon like peptide 1 (GLP-1), peptide tyrosine tyrosine (PYY) and oxyntomodulin are significantly elevated compared to preoperatively or to levels after AGB (e.g. [17, 23, 24]). Increased postprandial PYY and GLP-1 responses are observed from the early postoperative days after RYGB, prior to any significant weight loss [25]. Moreover, in a randomised double-blind controlled study of patients after RYGB and LAGB, inhibition of the gut hormone responses with octreotide, a somatostatin analogue that blocks the release of gut hormones, increased food intake in the RYGB group, but not in the LAGB group, suggesting that gut hormones play a role in the reduced food intake after RYGB but not after AGB [17]. Furthermore, PYY and GLP-1 responses correlate with different levels of weight loss post-RYGB; patients with 20 % weight loss had lower PYY and GLP-1 levels compared to patients that lost 40 % of their weight after surgery [17]. The exaggerated release of these anorexigenic hormones may be due to heightened stimulation of the small bowel L cells by undigested nutrients or nutrient sensing in the proximal small bowel that signals to the distal small bowel to release gut hormones [26].
What is intriguing is that postprandial GLP-1 and PYY responses after VSG (e.g. [27, 28]), which was originally thought to be a restrictive procedure, are comparable to RYGB and probably mediating the reduction in appetite observed postoperatively. The mechanism underlying this unexpected observation is not understood, but may be due to the rapid gastric emptying propelling nutrients to the distal small bowel and stimulating L cells [29]. The AGB is not associated with significant changes in the release of these anorexigenic gut hormones (e.g. [17, 23]).
Ghrelin is produced by the stomach and is the only known orexigenic hormone. Even though earlier studies showed that plasma levels are substantially decreased after RYGB, others have shown that it either remains stable or decreases (e.g. [30, 31]). These discrepancies may be due to the different laboratory handling of ghrelin samples, nutritional state of participants and whether total or active ghrelin is assayed [32]. The results appear to be more consistent for VSG and AGB, with decreases and increases in plasma ghrelin reported respectively [18, 33].
Plasma levels of leptin are lowered after any weight loss intervention in proportion to fat mass loss, and result in increases in the feeling of hunger (e.g. [19, 34]). However, even after substantial reductions of plasma leptin after all bariatric surgery procedures, rebound hyperphagia is not observed, suggesting that their other anorexigenic mechanisms might be enough to compensate for lower leptin levels.
More recently, a series of publications by one group has casted some doubt as to the physiological importance of alterations of gut hormone levels after VSG [35, 36]. In these studies, GLP-1 and ghrelin knockout rodent models of VSG reduced their weight and food intake similarly to the wild type animals. Whilst there is a need for these results to be replicated by other researchers, it may be the case that these knockout models develop compensatory mechanisms to compensate for their genetic defect, allowing them to behave like the wild type animals.
Neural Signalling After Bariatric Surgery
The vagal nerve plays an important role in the regulation of food intake and body weight [37]. Vagal afferents are activated by the presence of nutrients in the stomach and the intestine. The release of gut hormones, as well as mediation of their effects, is influenced by the functionality of the vagus. Indeed, the preservation of vagal fibres during surgery leads to greater and more sustained body weight loss in animal models of the RYGB [38]. Pressure generated in the proximal alimentary limb of the RYGB by a 20 mL balloon appears to predict the meal size of a patient [39]. Thus the rapid entry of food from the oesophagus, through the small gastric pouch and the gastro-jejunal stoma may trigger neural signals in the alimentary limb which may contribute to long-term weight loss maintenance [39].
Whilst it is still unknown as to whether the vagus contributes to weight loss after VSG, a few elegant experiments have shown that it may be the predominant mechanism after AGB. In a randomised controlled double-blind study in humans, optimal adjustment of the band through insertion of fluid was associated with reductions in hunger ratings before a meal and increases in satiation ratings after a meal, as compared to when the fluid was completely or partially removed from the band [18]. Additionally, acute and chronic inflation of the banding mechanism in rodents was accompanied by stimulation of vagal afferents and increased neuronal activity in the nucleus of the solitary track, a brainstem region involved in the control of food intake [40]. These findings suggest that the higher intraluminal pressure in the gastric fundus generated as a result of placement of the band itself, and further amplified by food intake, may trigger a cascade of signalling in the vagus–brainstem–hypothalamic axis resulting in decreased caloric intake and weight loss. The optimal pressure of 25–30 mmHg within the banding system is achieved through insertion of different amounts of fluid between patients, with exponential increase in pressure when too much fluid is inserted [41]. It is therefore crucial that adjustments are carefully made if the unwanted complication of mechanical restriction and vomiting are to be avoided.
Energy Expenditure
Chronic caloric deprivation is normally accompanied by a decrease in resting energy expenditure as the body strives to conserve energy [42]. These are some inconsistencies as to what happens to resting energy expenditure after bariatric surgery, but the majority of human and/or animal studies have shown that it remains stable on decreases after RYGB, VSG and AGB [21, 43–52]. However, a more consistent finding from both humans and rodents is that postprandial energy expenditure is higher after RYGB compared to controls [53–55]. Similar experiments have not been performed after VSG or AGB.
The postprandial energy expenditure increases after RYGB are mechanistically intriguing. Gut hormones may contribute to enhanced postprandial thermogenesis through centrally mediated sympathetic nervous system activation. Against this hypothesis, acute peripheral administration of exendin (9–39), a GLP-1 receptor antagonist did not alter energy expenditure in rodent models of RYGB [56]. An alternative hypothesis is that the elevation in the plasma levels of bile acids after RYGB may lead to heightened activation brown adipose tissue and consequently higher postprandial thermogenesis. Unfortunately, the only available rodent study failed to demonstrate this [57]. Therefore the mediators underlying this paradoxical increase in energy expenditure in the context of weight loss remain elusive, but have attracted considerable interest as they may be the targets of novel anti-obesity pharmacotherapy.
Gut Microbiota
The role of gut microbiota in the context of obesity and weight loss has attracted significant interest. Obesity is associated in some, but not all studies, with an unfavourable colonisation of the bowel with bacteria that are more efficient in extracting energy from nutrients and storing it as fat [58]. A profound disturbance of this colonisation has been observed after RYGB in particular and includes the reduction in Prevotellaceae, Archea, Firmicutes, Bacteroidetes and an increase in the Bacteroidetes/Prevotella ratio and Gammaproteobacteria [59–61]. These alterations may be due to weight loss itself, changes in macronutrient proportions in the diet, anatomical manipulations, pH and bile flow amongst others. The confounding effects of variations in these factors together with the antibiotic use and metabolic control may be the cause of the variability of the results in the handful of published studies. More recently, other novel mediators that may contribute to weight loss have surfaced. In a series of very elegant experiments, the transfer of gut microbiota from mice that have undergone RYGB to germ-free mice lead to 5 % weight loss in the latter group, potentially through altered short-chain fatty acid production and signalling [62].
Food Preferences
A number of human studies have reported that it is not only total caloric intake that is lower after RYGB, but also the percentage of calories from fat and sugar as compared to preoperatively or after other bariatric surgical techniques such as the vertical/horizontal banded gastroplasties [43, 63–69]. Similar to the energy expenditure observation, this finding is somewhat paradoxical considering that diet-induced weight loss is normally associated with more “cravings” for high-calorie, energy dense, fatty and sweet foods in the majority of cases (e.g. [20]). The human literature does suffer from some inconsistencies as to the magnitude and durability of this observation. Indeed, the study of human eating behaviour is notoriously difficult due to the variability of human nature itself and the imprecise methods of assessing it, which is further amplified by the stigma and underreporting of caloric intake associated with obesity. Nevertheless, the caveats of human eating behaviour research can be largely avoided through the use of animal models of RYGB. The relative consumption of fat and sugar is lower in rodents after RYGB compared to sham-operated rats, a finding consistent amongst different laboratories and with the majority of the human literature (e.g. [70, 71]).
The mechanisms responsible for this “healthy” shift in food preferences have attracted considerable interest in the last few years. The available literature suggests that the mechanism is multifactorial; patients after RYGB exhibit increased taste acuity for sweets [72], the appetitive reward value of fat/sweet taste is decreased [73] and in brain reward system activation in response to food and/or high-calorie food pictures, as assessed by functional neuroimaging, is lower after RYGB compared to preoperatively or after AGB (e.g. [74, 75]). These responses may be further amplified by unpleasant post-ingestive effects of energy dense food, in the form of the dumping syndrome and condition taste aversion (e.g. [70]). VSG is also associated with similar responses to food in rodent models of the procedure [76]. The mediators underlying the lower consumption of fat and sugar after RYGB and VSG have not been elucidated yet, but may include the action of gut hormones on taste afferents and the mesolimbic brain reward system, and altered nutrient sensing and vagal signalling in the gut.
Bariatric Surgery and Type 2 Diabetes Mellitus
Clinical Efficacy of Metabolic Surgery
Out of all the comorbidities associated with obesity, bariatric surgery is particularly effective in improving glycaemic control in patients with T2DM. A substantial proportion of these patients achieve euglycaemia, or even normoglycaemia, in the absence of active glucose-lowering pharmacotherapy [3–6]. The high rates of glycaemic “remission” after bariatric surgery have led to the adoption of the term “metabolic surgery” to describe its potent metabolic efficacy. In the absence of a consistent definition, a high-profile meta-analysis reported remission rates of 78 % of patients after surgery [77]. Subsequent analyses of different cohorts have shown that the remission rates are 34–41 % when the more stringent glycaemic criteria of the American Diabetes Association are applied [4, 78]. Recognising the need for more holistic outcomes, the International Diabetes Federation (IDF) introduced their own criteria of optimisation of the metabolic state which incorporate markers of glycaemia, but also weight loss, plasma lipids, rates of hypoglycaemia, blood pressure control and use of medications [79]. Using these criteria in a small cohort, remission rates of only 8–14 % were achieved 1–2 years after metabolic surgery, suggesting that surgery may be better used together with, and not instead of, lifestyle modification and pharmacological treatments for T2DM for optimal metabolic outcomes [80]. Independently of what criteria are used to define metabolic remission, the clinical efficacy of metabolic remains substantial and superior to currently available best medical care. This has now been consistently proven by four RCTs [3–6] and a number of others are ongoing.
Glycaemic improvements after surgery do not follow the same pattern after all metabolic procedures. The BPD, RYGB and VSG cause rapid reductions in blood glucose within days after surgery and before significant weight loss has been achieved, in contrast to the AGB after which the reductions are slower and gradual [81–83]. As the criteria used to define T2DM remission, change from study to study, it is very difficult to make definitive conclusions on the relative efficacy of each procedure. However, the available evidence suggest that BPD is superior to RYGB and VSG, with the latter two having very similar success rates in the medium term, followed by the AGB [3–5]. These results remain the same even when patients in each surgical group are matched for weight loss, suggesting that some of these procedures have weight-loss-independent effects [3, 25]. In this section, we discuss the mechanisms underlying the observations of these RCTs. These are also summarised in Table 10.1.
Mechanisms Underlying Metabolic Improvements
Beta-Cell Function
After RYGB, first phase and early insulin release is increased both early postoperatively and late after significant weight loss. This is supported by the vast majority (e.g. [34, 84–98]), but not all [99], of the studies that used oral glucose/mixed meal tolerance or intravenous tests. These have shown that both the glucose and insulin responses after an oral challenge are shifted to the left, as the result of faster glucose absorption and exaggerated and rapid insulin secretion. Indeed, after RYGB, the disposition index, acute insulin response to glucose and the insulinogenic index increase in patients with T2DM, but remain stable or may even be reduced in subjects with normal glucose tolerance [94, 95, 97, 98]. Additionally, both the beta-cell sensitivity is increased and the proinsulin/insulin ratio decreases after RYGB [84, 96, 100]. As would be expected in response to weight loss and improvements in peripheral insulin resistance, the decline in insulin release after a meal is rapid, and the total postprandial area under the curve (AUC) for insulin is lower compared to preoperatively [86, 88, 89, 91, 101]. When patients after RYGB and AGB are studied following 20 % weight loss, total postprandial AUC is reduced similarly by these procedures [102]. However, the insulin curves look very different, with a characteristic earlier and greater insulin peak after RYGB, but not AGB [102]. The responses after VSG are similar to those observed after RYGB (e.g. [28, 103, 104]). The BPD is unique in that it profoundly reduces both hepatic and peripheral insulin resistance very early after surgery and before significant weight loss has taken place, but also increases early insulin secretion in patients with T2DM, but not normoglycaemic patients (e.g. [105, 106]). Again following weight loss after BPD, total postprandial AUC is substantially decreased compared to preoperatively [107].
The mechanism underlying the increased early postprandial release of insulin after RYGB is in part due to the rapid and exaggerated rise in the incretin hormone GLP-1, as administration of the GLP-1 receptor antagonist exendin (9–39) attenuates this response [108]. Whilst such mechanistic studies have not been performed after BPD, similar results to RYGB are observed after VSG in animal models [109].
There is however another side to the reversal of beta-cell dysfunction after RYGB and this is postprandial hyperinsulinaemic hypoglycaemia. In the largest patient cohort even examined through a national registry in Sweden, the relative risk of hypoglycaemia was two- to sevenfold higher after RYGB, but not VBG or AGB, compared to the general population [110]. However, the absolute rates of symptomatic hypoglycaemia remain low at 0.2–1 %.
There is controversy as to the mechanism underlying this phenomenon with some studies suggesting it is the result of beta-cell hyperplasia (e.g. [111]), whereas others supporting that it is due to “extreme” restoration of beta-cell function and GLP-1 release (e.g. [112]). Hyperinsulinaemic hypoglycaemia can be treated through dietary modification and avoidance of foods with a high glycaemic index. Pharmacological therapies include acarbose, somatostatin analogues and diazoxide. The last resort, in the rare unresponsive cases, involves reduction in beta-cell mass through a partial pancreatectomy.
Insulin Resistance
The RYGB, AGB and VSG procedures cause reductions in peripheral insulin resistance directly as a result of gradual weight loss [81]. However, in some human studies, hepatic insulin resistance is reduced within days after RYGB before any clinically significant weight loss has taken place (e.g. [25, 113]). Whilst this may be a direct result of a reduction in caloric intake [114], the reduction in hepatic insulin resistance in some studies is greater in patients after RYGB compared to patients undergoing similar caloric restriction after AGB or a hypocaloric diet [25, 84, 113
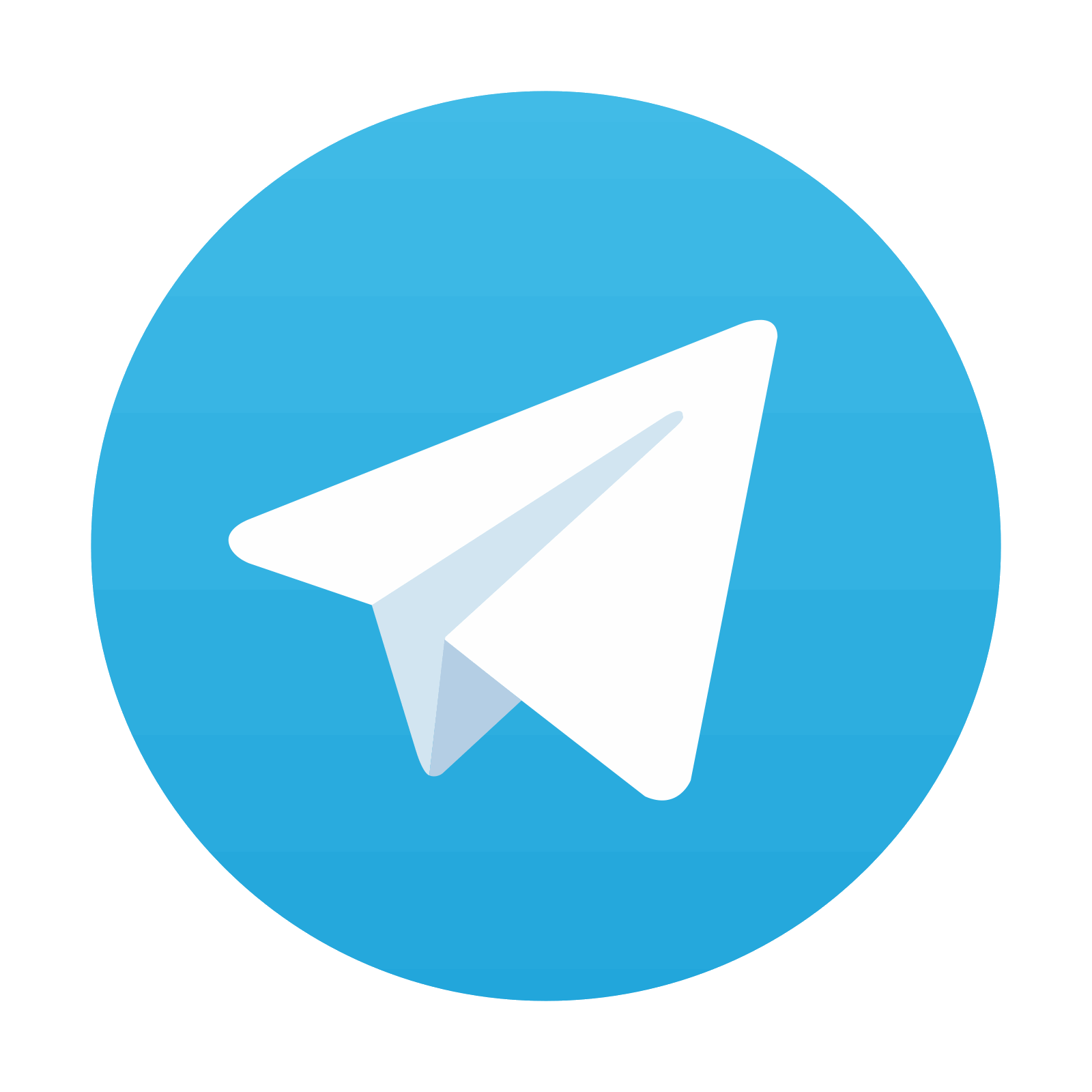
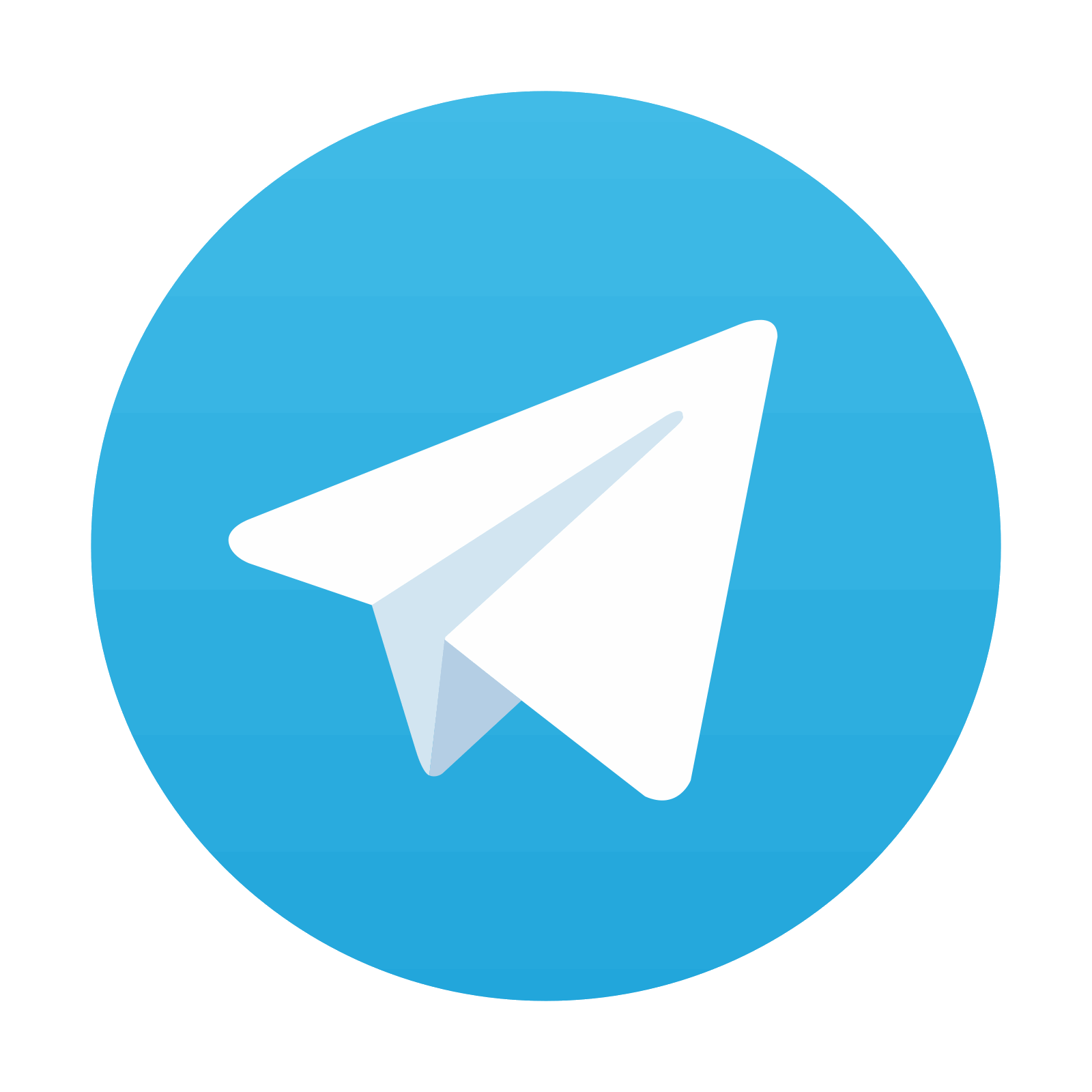
Stay updated, free articles. Join our Telegram channel
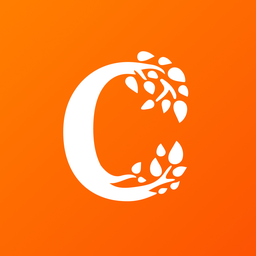
Full access? Get Clinical Tree
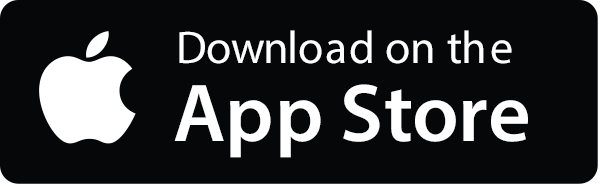
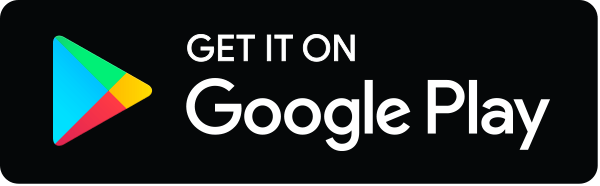