1.Light represents one portion of a broader electromagnetic spectrum.
2.Light-tissue interactions involve the complex topics of tissue optics, absorption, heat generation, and heat diffusion
3.Lasers are a special type of light with the characteristics of monochromaticity, directionality, and coherence.
4.Coagulation/denaturation is time and temperature dependent
5.Proper selection of light parameters is based on the color, size, and geometry of the target
6.Wound healing is the final but not least important part of the laser tissue sequence (the epilogue)
7.Laser-tissue interactions are fluid – the operator should closely examine the skin surface during all aspects of the procedure
8.Pulse duration and light doses are often as important as wavelength in predicting tissue responses to laser to irradiation
The best gauge of laser interactions is the tissue response, and experiment is the most realistic manner to address medical treatment challenges. However, theoretical models are helpful in planning treatment approaches and laser parameters. In this chapter we discuss basics of lasers, their non-laser counterparts, and laser-tissue interactions.1
Many physicians choose laser settings out of habit (or reading it off of a label attached to the side of the machine – a “cheat” sheet with skin-type specific parameters), using tissue endpoints to confirm the appropriateness of the parameters. For example, when treating a tattoo with a Q-switched laser, the operator looks for immediate frosty whitening. Like driving a car (where the operator may have no idea about nature of the drive train components), successful laser operation does not demand a complete understanding of the machine or the details of the light-tissue interaction. However, a comprehension of first principles allows for a logical analysis of final clinical outcomes – furthermore, more creative uses of equipment should follow. For example, with an education in laser tissue interactions (LTIs) and tissue cooling, one can deploy the alexandrite (long pulse) laser either as a hair removal device, vascular laser, or to remove lentigines.2
Light
Light represents one portion of a much broader electromagnetic spectrum. Light can be divided into the UV (200–400 nm), VIS (400–700 nm), NIR “I” (755–810 nm), NIR “II” (940–1,064 nm), MIR (1.3–3 μm), and Far IR (3 μm and beyond). On a macroscopic level, light is adequately characterized as waves. The amplitude of the wave is perpendicular to the propagation direction. Light waves behave according to our “eyeball” observations in day-to-day life. For example, we are familiar with refraction and reflection. The surface of a pond is a partial mirror (reflection); a fish seen in the pond is actually closer than it appears (refraction).3 Normally, the percentage of incident light reflected from the skin surface is determined by the index of refraction difference between the skin surface (stratum corneum n = 1.55) and air (n = 1).4 This regular reflectance is about 4–7% for light incident at right angles to the skin.3,5 The angle between the light beam and the skin surface determines the % of reflected light. More light is reflected at “grazing” angles of incidence. It follows that, to minimize surface losses, in most laser applications, one should deliver light approximately perpendicular to the skin.3,6 One can deliberately angle the beam, on the other hand, to decrease penetration depth and also attenuate the surface fluence by “spreading” the beam. One can reduce interface losses by applying an alcohol solution (n = 1.4), water (n = 1.33), or a sapphire crystal (n = 1.55 μm). This allows for optical coupling (vide infra). On the other hand, the surface of dry skin reflects more light because of multiple skin–air interfaces (hence the white appearance of a psoriasis plaque).
Light penetrates into the epidermis according to wavelength dependent absorption and scattering (vide infra).1,6–8 Because of scattering, much incident light is remitted (remittance refers to the total light returned to the environment due to multiple scattering in the epidermis and dermis, as well as the regular reflection from the surface). In laser surgery, light reflected from the surface is typically “wasted”. This “lost” energy varies from 15% to as much as 70% depending on wavelength and skin type. For example, for 1,064 nm, 60% of an incident laser beam may be remitted. One can easily verify this by holding a finger just adjacent to the beam near the skin surface. Warmth can be felt from the remitted portion of the beam.
To describe laser tissue interactions at the molecular/microscopic level, light is considered as a stream of “particles” called photons, where the photon energy depends on the wavelength of light.


(1)
Where h is Plank’s constant (6.6 × 10−34 J-s), and c is the speed of light (3 × 1010 cm/s).9
Types of Light Devices
In principle, many non-laser devices could be used for heating skin.9 Most properties of laser light (i.e., coherence) are unimportant insofar as the way light interacts with tissue in therapeutic applications. And although collimation (lack of divergence) of the incident beam might increase the % of transmitted light with laser versus IPL, the increasing use of filtered flash lamps in dermatology suggests that losses from IPL beam divergence are not critical. In lieu of lasers, some thermal sources can be used in skin surgery (i.e., nitrogen plasma device) for resurfacing (Portrait, Rhytec, MA). The critical features of any device are controlling the device-tissue interaction time to allow for precise heating (vide infra).
Lasers are useful because they allow for precise control of where and how much one heats.10 There are four properties that are common to all laser types (1) Beam directionality (collimation), (2) Monochromaticity, (3) Spatial and temporal coherence of the beam, and (4) High intensity of the beam.11 The intensity, directionality, and monochromaticity of laser light allow the beam to be expanded, or focused quite easily. With non-laser sources like flashlamps directed toward the skin surface, the light intensity at the skin surface cannot exceed the brightness of the source lamp. With many lasers, a lamp similar to the intense pulsed light (IPL) flashlamp pumps the laser cavity.12 The amplification of light within the laser cavity sets laser light apart from other sources.
For most visible light applications, laser represents a conversion from lamplight to an amplified monochromatic form.13 The high power possible with lasers (especially peak power) is achieved through resonance in the laser cavity. For many dermatology applications requiring ms or longer pulses delivered to large skin areas, IPLs are either adequate or preferable to lasers. The scientific principle on which lasers are based is stimulated emission. With spontaneous emission, electrons transition to the lower level in a random process. With stimulated emission, the emission occurs only in the presence of photons of a certain change. The critical point is maintaining a condition where the population of photons in a higher state is larger than that in the lower state. To create this population inversion, a pumping energy must be directed either with electricity, light, or chemical energy.
All lasers contain four main components, the lasing medium, the excitation source, feedback apparatus, and an output coupler. With respect to lasing media, there are diode lasers, solid-state lasers, dye, and gas lasers. Most solid state and dye lasers use optical exciters (lamps), whereas gas and diode lasers use electrical excitation (i.e., CO2 and RF). The feedback mechanism consists of mirrors where one mirror reflects 100% and the other transmits a small fraction of light.14 An example of a solid-state laser is the alexandrite laser. A solid-state laser consists of a rod that is pumped by a flashlamp. The lamp pumps the rod for stimulated emission. The rod and lamp assembly must also be designed for adequate cooling. Lasers typically are finicky because all of the components are driven near their damage thresholds (like redlining your car all the time). As an example of this concept, consider the pulsed dye laser (PDL). As the dye degrades, the lamps must work harder to generate higher pulse energies from the dye. Also, mirrors become contaminated over time such that the lamps must work harder and harder. These demands stress the power supply. Thus, eventually, the dye kit, the power supply, lamps, and dye are all working at their maximal output. Often people speak of a tunable dye laser. In fact many dye lasers are tunable; the manufacturers have simply chosen one wavelength. An example of a tunable laser was the Scleroplus pulsed dye laser (tunable from 585 to 600 nm in 5 nm increments) from Candela (Candela, Wayland, MA).
Laser systems differ with regard to duration and power of the emitted laser radiation. In continuous wave lasers (CW mode) with power outputs of up to 103 W, the lasing medium is excited continuously. With pulsed lasers, excitation is effected in a single pulse or in on-line pulses (free-running mode). Peak powers of 105 W can be developed for a duration of 100 μs–10 ms. Storing the excitation energy and releasing it suddenly (Q-switch mode or mode-locking) leads to a peak power increase of up to 1010–1012 W, and a pulse duration of 10 ps–100 ns.13
Light emitting diodes (LEDs) are becoming commonplace in dermatology (Fig. 1). Primarily used as a PDT light source, they are also used in biostimulation. LEDs are similar to semiconductor (aka diode) lasers in that they use electrical current placed between two types of semiconductors. However, they lack an amplification process (no mirrors). LEDs do not produce coherent beams but can produce monochromatic light. Semiconductor (diode) lasers contain an LED as the active gain medium. A current passes through a sandwich of two layers consisting of compounds (called p type and n type). Below threshold, there is no oscillation and the semiconductor LASER acts like an LED. This emission is very similar to the visible emission of light emitting diodes. If one adds mirrors it operates as a tiny laser instead of an LED. The overall efficiency of semiconductor lasers is quite high, approximately 30% and among the highest available. Most semiconductor (diode) lasers are operated in CW mode but can be pulsed. New visible semiconductor lasers are available and also laser diode arrays are available where scientists have created large numbers of semiconductor lasers on one substrate. Some diode lasers are housed separate from the handpiece and delivered by fiber optics. Others are configured with the laser diodes in the handpiece as arrays. Modern diode lasers achieve higher powers than in the past, but their peak powers are still lag behind most pulsed solid-state lasers.14
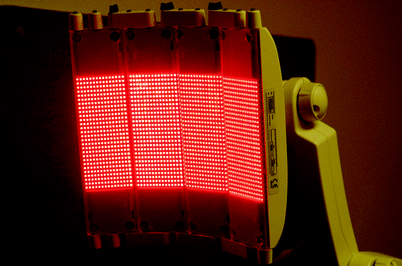
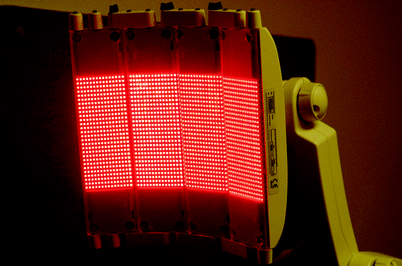
Fig. 1
A red LED (OmniLux, Phototherapeutics, Inc.)
Excimer lasers emit UV light and are used for photomodulation of the immune system. They have also been used in surgery. The possible mutagenicity of these lasers has not been well studied. Materials such as the KTP crystal can be used to generate harmonics with lasers. The KTP crystal is used to convert 1,064 nm radiation to 532 green light. Also quality (Q) switching is used for generating short pulses. Much of the electrical energy used to create laser emissions is wasted as heat, which is why water is used for cooling most lasers. Air cooling is used for some high-powered flash lamps and many diode lasers. In the future, free electron lasers might be useful but presently they are too cumbersome and only generate small amounts of energy per unit wavelength.
Intense pulsed light devices are becoming increasingly comparable to lasers that emit ms domain pulses.15 Absorption spectra of skin chromophores show multiple peaks (HgB) or can be broad (melanin),16 and therefore a broadband light source is a logical alternative to lasers. Proper filtration of a xenon lamp tailors the output spectrum for a particular application. Some concessions are made with direct use of lamplight. For example, rapid beam divergence obliges that the lamp source be near the skin surface. This subsequent requirement makes for a typically heavier handpiece compared with most lasers (Fig. 2) (the exception being some diode arrays where the light source is also housed in the handpiece-(i.e., Light Sheer, Lumenis, CA)). Also IPL cannot be adapted to fibers for subsurface delivery. High energy short pulses (Q–switched ns pulses) are not possible with flashlamps. They can, however, be used to pump a laser, and some modern IPLs feature a laser attachment where the flashlamp and laser rod are in the handpiece. In general, the size, weight, and cost of both laser and flashlamp technology are steadily decreasing.
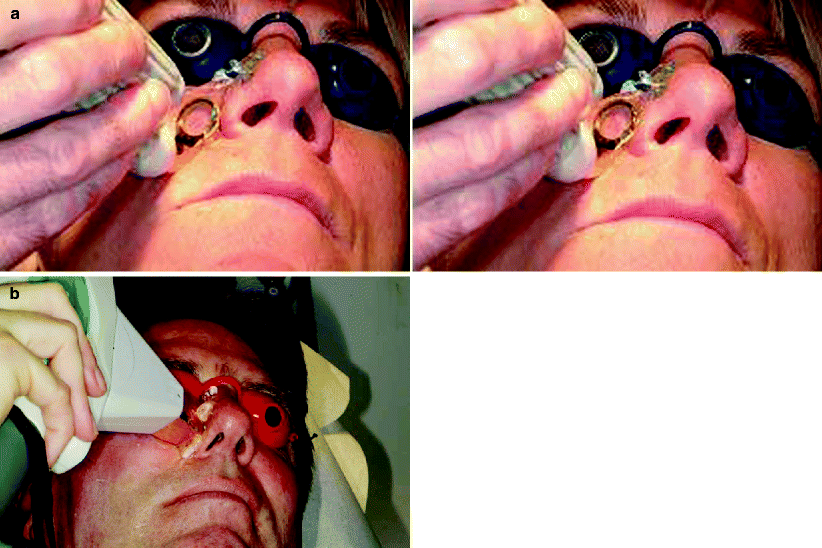
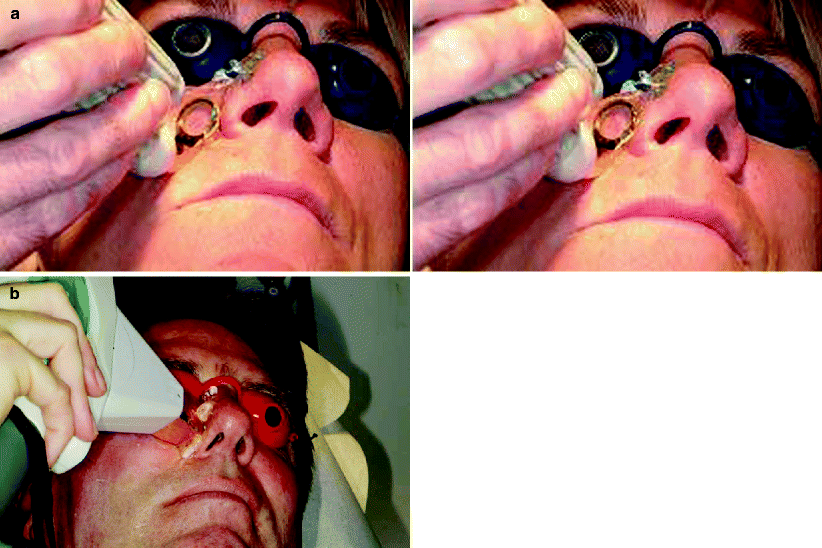
Fig. 2
IPL and green light laser – note smaller size of laser handpiece
Light Device Terminology
Basic parameters for light sources are power, time, and spot size for continuous wave lasers, and for pulsed sources, the energy per pulse, pulse duration, spot size, fluence, repetition rate, and the total number of pulses.17 Energy is measured in joules (J). The amount of energy delivered per unit area is the fluence, sometimes called the dose or radiant exposure, given in J/cm2. The rate of energy delivery is called power, measured in watts (W). One watt is one joule per second (W = J/s). The power delivered per unit area is called the irradiance or power density, usually given in W/cm2. Laser exposure duration (called pulsewidth for pulsed lasers) is the time over which energy is delivered. Fluence is equal to the irradiance times the exposure duration.10 Power density is a critical parameter, for it often determines the action mechanism in cutaneous applications (Fig. 3). For example, a very low irradiance emission (typical range of 2–10 mW/cm2) does not heat tissue and is associated with diagnostic applications, photochemical processes, and biostimulation. On the other extreme, a very short ns pulse can generate high peak power densities associated with shock waves and even plasma formation.19 Plasma is a “spark” due to ionization of matter.
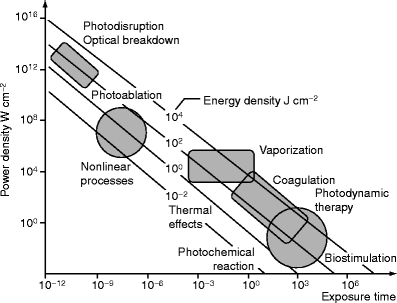
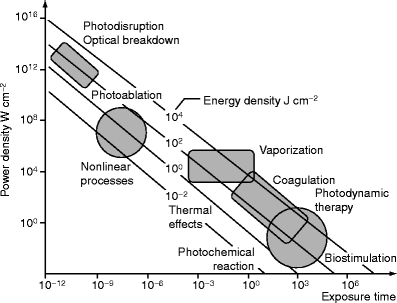
Fig. 3
Different types of laser tissue interactions depend on power density and exposure (From Knappe et al.18; Fig. 2)
Another factor is the laser exposure spot size (which greatly affects the beam strength inside the skin). Other characteristics of importance are whether the incident light is convergent, divergent, or diffuse, and the uniformity of irradiance over the exposure area (spatial beam profile). The pulse profile, that is, the character of the pulse shapes in time (instantaneous power versus time) also affects the tissue response.20
Many lasers in dermatology are pulsed, and the user interface shows pulse duration, fluence, spot size and fluence. Some multi-wavelength lasers also allow for wavelength selection. Some older lasers, for example a popular CO2 laser, showed only the pulse energy on the instrument panel, or in continuous wave (CW) mode, the number of watts. In these cases one uses the exposure area and exposure time to calculate the total light dose (fluence).


(2)
With the exception of PDT sources and CW CO2 lasers, most aesthetic lasers create pulsed light. In many CW applications (i.e., wart treatment with a CO2 laser), the fluence is not of great importance in characterizing the overall tissue effect. A more important parameter is power density (where higher power densities achieve ablation and lower power densities cause charring), and the physician stops the procedure when an appropriate endpoint is reached. On the other hand, in PDT applications with CW light where the clinical endpoint might be delayed, the total fluence and power density are important predictors of the tissue response.
In CW mode, CO2 lasers are used with a focusing (non-collimated) handpiece such that the physician can control spot size and tissue effects simply by moving the handpiece tip toward or away from the skin. The subsequent rapid changes in power density offer “on the fly” flexibility and control.
A thorough knowledge of a specific laser’s operation and quirks is imperative for optimal and “safe” lasering. Vendors are creating lasers that are more intuitive to operate. Increasingly, manufacturers have added touch screen interfaces with application-driven menus and skin-type specific preset parameters. Some devices permit patient laser parameters to be stored for future reference. Most lasers are designed such that the handpiece and instrument panels are electronically interfaced. It follows that the laser control module “knows” what spot size is being used. Typically this “handshake” occurs when one inserts the handpiece into the calibration port, or through a control cable from the handpiece to the laser “main frame”. With others, one selects the spotsize on the display, and the laser calculates the fluence accordingly. For example, one of our erbium YAG lasers possesses interchangeable lenses for 1, 3, 5, and 7 mm spots. However, there is no feedback from the handpiece to the laser control board. The user “tells” the laser which lens cell is inserted, and the laser calculates the fluence based on the selected spot and selected pulse energy. In this case, if one changes the spot size (for example, by exchanging the 7 mm for the 3 mm lens cell), the laser still “thinks” the 7 mm spot is being used, and the actual surface fluence is now ∼ 5X the fluence on the panel. The resulting impact on the skin surface (the wound depth and diameter) should alert the enlightened user to reassess his parameter selection.
Most lasers calibrate through a system where the end of the handpiece is placed in a portal on the base unit (Fig. 4). This configuration allows for interrogation of the entire system, from the “pumping” lamps to the fiber/articulated arm to the handpiece optics. For example, if a fiber is damaged, the laser will fail calibration, and an error message appears. Other systems measure the output within the distal end of the handpiece using a small calibration module that “picks off” a portion of the beam.
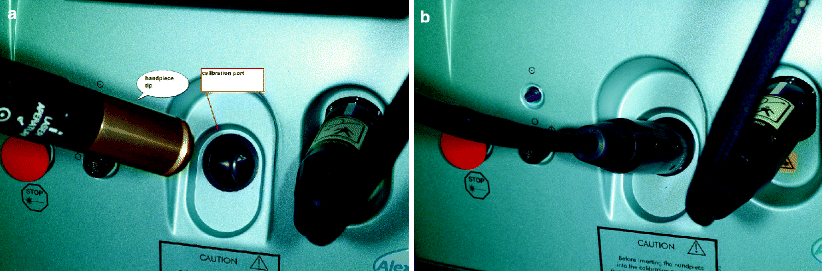
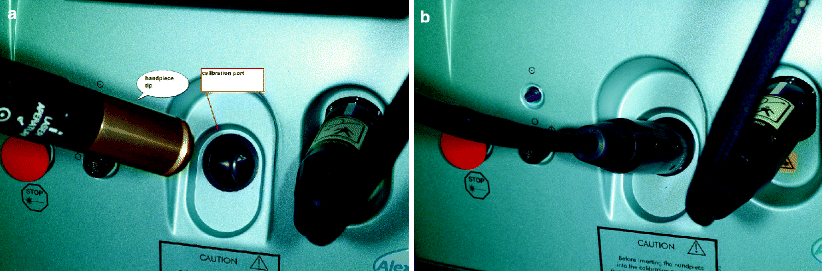
Fig. 4
Figures show handpiece before and during insertion into calibration port of a Q switched alexandrite laser
There are some simple ways to interrogate for system integrity. One can examine the aiming beam as it illuminates a piece of white paper, checking that the beam edges are sharp – this suggests that the treatment beam is also sharp and the profile is according to the manufacturer’s specifications. Also, burn paper can be used – here the laser is used with a low energy and the spot is checked for uniformity from beam edge to edge. By checking the impact pattern, one can uncover damaged mirrors in the knuckle of the articulated arm, or a damaged focusing lens that renders the laser unstable or unsafe. Likewise, for scanners, one can ensure that skin coverage will be uniform.
1.LEDs are becoming commonplace in biomedical applications 2.Solid state lasers generally achieve the largest peak powers among laser types 3.The laser operator should know every nook and cranny of a laser’s features to optimize patient outcomes and safety 4.Power density determines the mechanism for many LTIs |
Beam Profiles: Top Hat Versus Gaussian
Laser beam profiles vary based on intercavity design, lasing medium, and the delivery system. A common profile is Gaussian or bell-shaped. For many lasers, this profile represents the fundamental optimized “mode” of the laser. This shape is usually observed when the beam has been delivered through an articulated arm. For some wavelengths, this is an effective way to deliver energy (CO2 and erbium). The disadvantage of the rigid arm is limited flexibility, the typically short arm length, the possibility of misalignment from even minor impact, and a tendency for non-uniform heating across the spot.21 For example, in treating a lentigo with a Q-switched alexandrite laser equipped with a rigid articulated arm, one may observe complete ablation of the epidermis at the center of the “spot”, but only whitening at the periphery. On the other hand, sometimes a bell-shaped profile is desirable, for example, when applying a small spot FIR beam with a scanner. In this scenario, the wings of the beam allows for some overlap without delivering “too much” energy at points of overlap.
The Gaussian profile can be modified outside the cavity, which is desirable in many applications. With a fiber equipped delivery system, the beam is mixed within the fiber and can be shaped to be more flat-topped. The lentigo then is more likely to be uniformly heated (so long as the lesion itself if uniformly colored!). Although fiber delivery systems are usually preferred by physicians, some laser beams are difficult to deliver through a fiber. Examples include far IR wavelengths and very short pulses (i.e., few ns with typical Q switched Nd YAG lasers whose high peak power exceeds the damage threshold of most fibers).
Pulse Profiles: Square Versus Spiky
The pulse profile is the temporal shape of the laser pulse (Fig. 5).22 In many pulsed laser applications, the “macro pulse” is comprised of several shorter micropulses.23 Depending on the application, the temporal pulse profile may impact the tissue effect. For example, simply by increasing the pulse number from four to six pulselets, the purpura threshold is increased with the PDL. Also, highly energetic spikes tend to increase the epidermal to dermal damage ratio in applications such as laser hair reduction. This is especially true with green-yellow light in vascular applications.
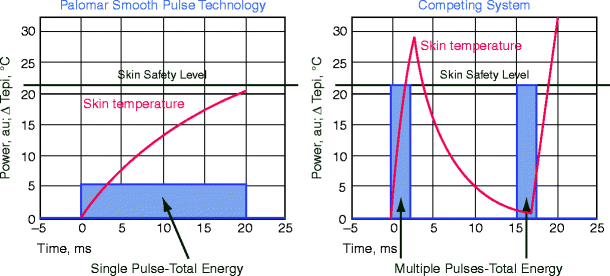
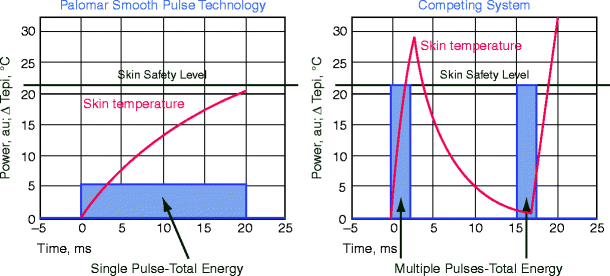
Fig. 5
Figure shows spiky versus smooth pulse and effect on epidermal temperature
Summary of Wavelength Ranges
In this section we examine wavelength ranges that are useful for cutaneous surgery.
1.
UV laser and light sources have been used primarily for treatment of inflammatory skin diseases and/or vitiligo, as well as striae. The presumed action is immunomodulatory. The XeCl excimer laser emits at 308 nm, near the peak action spectrum for psoriasis. Other UV non-laser sources have also been used for hypopigmentation, striae, and various inflammatory diseases.23,24 Excimer lasers at 193 nm have been used for skin and corneal ablation.
2.
Violet IPL emissions, low power 410 nm LED, and fluorescent lamps are used either alone or with ALA. Alone, the devices take advantage of endogenous porphyrins and kill P. acnes. 25 After application, of ALA, this wavelength range is highly effective in creating singlet O2 after absorption by PpIX. Uses include treatment of actinic keratoses, actinic cheilitis, and basal cell carcinomas.26
3.
VIS (GY). These wavelengths are highly absorbed by HgB and melanin and are especially useful in treating epidermal pigmented lesions and superficial vessels.27–29 Their relatively poor penetration in skin (and the even poorer penetration in blood – see Table 1) make them poor choices for treatment of deeper pigmented lesions or deeper larger vessels. Their shallow penetration depths preclude their use in permanent hair reduction (with the possible exception of very large spots (i.e., IPL) that enhance light depth). The effective portions of many IPL spectra include the GY range.
Table 1
Absorption coefficients (cm−1) for various chromophores
Wavelength (nm) | 410 | 532 | 595 | 694 | 755 | 810 | 940 | 1,064 |
Oxy HgB (40% Hct) | 1,990 | 187 | 35 | 1.2 | 2.3 | 3.6 | 5.2 | 2.2 |
Deoxy HgB | 1,296 | 138 | 96 | 6.6 | 5.2 | 2.7 | 3.0 | 0.6 |
Melanina | 140 | 56 | 38 | 23 | 17 | 13 | 7 | 5.7 |
Water | 6.7 × 10−5 | 0.00044 | 0.0017 | 0.005 | 0.03 | 0.02 | 0.27 | 0.15 |
Bloodless dermis | 10 | 3 | 2 | 1.2 | 0.8 | 0.6 | 0.5 | 0.4 |
OPD in skin (μm) | 100 | 350 | 550 | 750 | 1,000 | 1,200 | 1,500 | 1,700 |
By the proper manipulation a laser delivery device, one can optimize parameters for selective heating of pigmented versus vascular lesions. For example, by applying a compression handpiece without cooling with 595 nm, blood is depleted as a target and pigment is preferentially heated.31 Also, by (see limelight desert mode – Fig. 6), one can increase or decrease the sapphire window temperature to enhance epidermal versus vascular heating. By reducing the pulsewidth into the nanosecond range, melanosomes are preferentially heated over vessels. For example, extremely short Q-switched 532 nm pulses will cause fine vessels to rupture, but inadequate heat diffusion to the vessel wall precludes long term vessel destruction. On the other hand, melanosomes are sufficiently heated for single-session lentigo destruction. By choosing specific wavelengths with respect to HgB and melanin, one can achieve some degree of selective melanin or HgB heating. For example, if one wanted to avoid HgB in heating a lentigo, 694 nm (ruby) represents a better choice than 532 or 595 nm. This choice might decrease inflammation by unintended heating of normal vessels in the dermis.
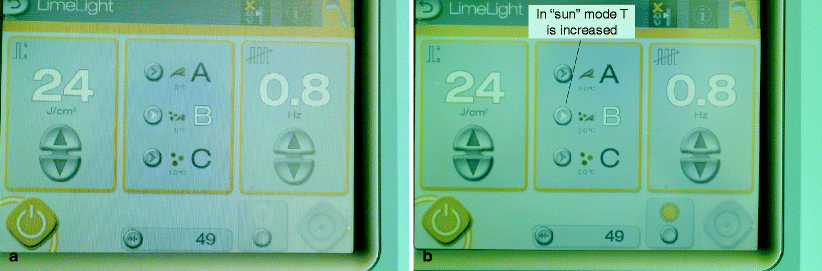
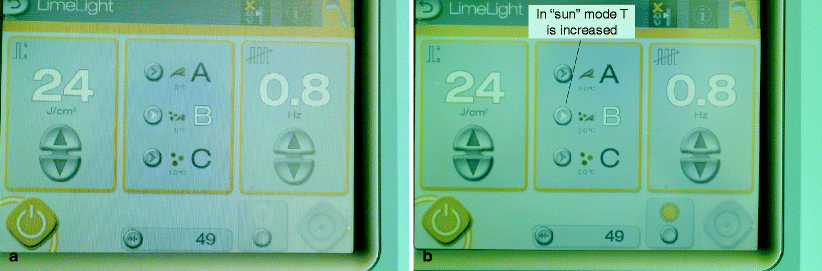
Fig. 6
Figure shows user controllable temperature change with an IPL. By increasing the handpiece tip temperature, pigmented lesion heating is favored over vascular heating
There are absorption peaks for PpIX in the green-yellow range, making these wavelengths useful for PDT (i.e., sodium lamp, IPL, frequency doubled Nd YAG, or PDL).32,33 On the other hand, all visible light can be used for PDT, as the Soret band and smaller “Q-bands” can all create singlet O2 on irradiation of PpIX. Therefore the 532, 595, and IPL devices, when used adjunctively with ALA, can all augment the cosmetic result through both photothermal and photochemical effects.
4.
Red and Near IR (I) (630, 694, 755, 810 nm). Deeply penetrating red light (630 nm) continuous wave devices are efficient activators of PpIX after topical application of ALA. The 694 nm (ruby) laser is optimized for pigment reduction and hair reduction in lighter skin types. The 810 nm diode and 755 nm alexandrite laser, depending on spot size, cooling, pulse duration, and fluence can be configured to optimize outcomes for hair reduction, lentigines, or blood vessels.34 They are positioned in the absorption spectrum for blood and melanin between the GY wavelengths and 1,064 nm. They will penetrate deeply enough in blood to coagulate vessels up to 2 mm35–37; also, they are reasonably tolerant of epidermal pigment in hair reduction (with surface cooling) so long as very dark skin is not treated.38 By decreasing the pulsewidth into the nanosecond range, the alexandrite laser is a first line treatment for many tattoo colors.
5.
Near IR (II). 940 and Nd YAG (1,064 nm). These two wavelengths have been used for a broad range of vessel sizes on the leg and face.39 They occupy a unique place in the absorption spectrum of our “big 3” chromophores, that is blood, melanin, and water. Because of the depth of penetration (on the order of mm), they are especially useful for hair reduction and coagulation of deeper blood vessels. By varying fluence and spot size, reticular ectatic veins, as well as those associated with nodular port wine stains or hemangiomas, can be safely targeted. On the other hand, they are not well suited for epidermal-pigmented lesions. Also, although water absorption is poor, it exceeds that of the VIS and near VIS wavelengths. The result is that 940 and 1,064 nm achieve large volume temperature elevation in the skin, and with repeated laser impacts, because of the slow cooling of this volume (large τ – vide infra), catastrophic pan-cutaneous thermal damage is possible. This wavelength (1,064 nm) represents the extreme example of a “what you don’t see can hurt you laser”19 (Fig. 7). The Q-switched YAG laser plays an expanding role in the treatment of tattoos, nevus of Ota, and even melasma.
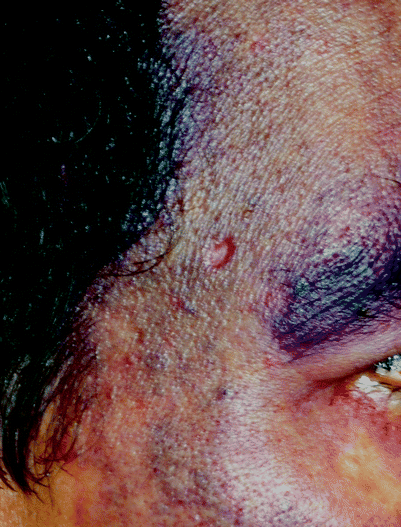
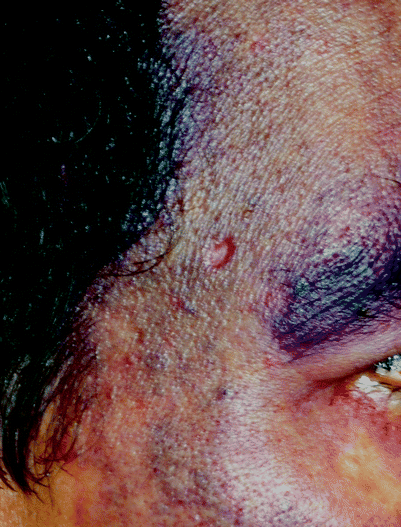
Fig. 7
Note scar after 1,064 nm irradiation of a nodule within a port wine stain
6.
MIR-lasers and deeply penetrating halogen lamps. These lasers and lamps heat tissue water. With macrowounding (>1 mm) spot sizes, depending on where we want to heat, we can “choreograph” our laser and/or cooling settings to maximize the skin temperature in certain skin layers. In general, with more deeply penetrating wavelengths, larger volumes are heated. On the other hand, achieving temperature elevations in the volume will require higher fluences than with highly absorbing wavelengths. Without surface cooling, unless very small fluences are applied, a top to bottom thermal injury occurs. The absorption coefficients for the 1,320, 1,450, and 1,540 nm systems are ∼3, 20, and 8 cm−1 respectively,40 while the effective scattering coefficients are about 14, 12, and 11 cm−1. The corresponding penetration depths are ∼1,500, 300, and 700 μm. It follows that for equal surface cooling and equal fluences, the most superficial heating will occur with the 1,450 nm laser, followed by the 1,540 and 1,320 nm lasers. Newer deeply penetrating lamps have been introduced (Titan, Cutera, Brisbane, CA). They emit light over a 1–2 μm wavelength band with relatively low power densities and long exposures (several seconds). In a typical scenario, the irradiation begins after a roughly two second period of cooling. At this point, a band of tissue from roughly 700 μm–1.5 mm deep in the skin is heated. By varying the fluence, this relatively large volume can be heated to different peak temperatures. As part of each iteration, post pulse cooling is imperative because such a large volume of skin is heated that a “thermal wake” advances toward the skin surface. If one removes the handpiece prematurely, heat accumulates near the skin surface with the risks of pain, dermal thermal injury, and scarring.41 The 1,320 nm Nd:YAG has been used in the endovenous ablation of the deep saphenous venous system as well as laser liposculpture. Recently the MIR spectral subset has become the mainstay for fractional non-ablative technologies.
7.
Far infrared systems. The major lasers are the CO2, erbium YAG, and erbium YSGG (chromium:yttrium-scandium-gallium-garnet) lasers. Overall, the ratio of ablation to heating is much higher with the erbium YAG laser. However, one can enhance the thermal effects of the Er YAG laser by extending the pulse or increasing the repetition rate, and likewise one can decrease residual thermal damage (RTD) of the CO2 laser by decreasing pw.42,43 Where precision is required in ablation, Er YAG is preferred. On the other hand, depending on settings, the CO2 laser enjoys a desirable blend of ablation and heating. The thresholds for ablation for CO2 and erbium lasers vary inversely with their optical penetration depths in tissue (20 μm and 1 μm respectively). This assumes thermal confinement. It follows that less surface fluence is required for ablation with the erbium laser. The CO2 laser at typical operating “pulsed” parameters performs self-limited controlled heating of the skin,44,45 whereas the erbium laser operates in an almost purely ablative regime. The erbium YSGG (2.79 μm) laser has recently been applied to LSR and its absorption coefficient makes it a kind of hybrid between CO2 and erbium YAG insofar as the ratios of heating to ablation. All three wavelengths (2.79, 2.94, and 10.6 μm) have recently been integrated into fractional delivery systems.
Radiofrequency (RF) Technology
With Radio frequency energy, local heat generation depends on the local electrical resistance and current density. The distribution of the current density is determined by the configuration of the electrodes with respect to the skin anatomy. There are two types of electrode deployments.46–49 In one scenario, cooled bipolar electrodes are combined with a diode laser, halogen lamp, or intense pulsed light device. In this configuration, there is synergy between the two energy sources.50 With the bipolar electrode configuration confines the electrical field density is superficially (the field intensity reaches about as deep as one half the distance between the electrodes).
In monopolar configurations, a smaller square electrode placed over the skin “target” is combined with a larger the dispersive electrode is located at a distant point on the body. Monopolar skin rejuvenation systems create large-volume heating. Electrical energy is distributed uniformly over the electrode surface through “capacitive coupling”. This type of coupling reduces the natural accumulation of electrical energy at the electrode edge.51 The first non-ablative RF device (Therma Cool TC, Thermage, Hayward, CA) uses cryogen spray cooling (CSC), where the spray is started before the RF current.
If both positive and negative electrodes are placed in the contact tip (bipolar electrode), current density tends to flow superficially (path of least resistance from electrode to electrode, and therefore temperature elevation is confined to superficial skin). By placing the electrodes further apart, the current density depth will increase. Otherwise, control of the tissue heating is determined by variations in electrode type, power, and cooling times.50 If one uses “rail” metal type electrodes placed next to a flashlamp crystal, EM field theory predicts that there will be a hot spot near the edge of the electrode. These hot spots can be reduced by electrically coupling the energy into the skin (for example, ensuring that the dry stratum corneum, with high intrinsic impedance, is wetted with an electrolyte solution).
One representative bipolar device (Polaris, Syneron, Richmond Hill, Ontario, Canada) combines RF energy and a 980 diode laser. In this configuration, the local optical energy (fluence) increases the discrete chromophore temperature (i.e., hair, vessel). Localized heating reduces impedance (skin is treated as an electrolyte with decreasing impedance as a function of increasing temperature) and therefore in higher localized current densities. Thus, there is “synergy” between the optical and electrical parts of the device.50,52–54 A purported advantage of the treatment is that lower optical energies can be used to selectively heat sub-surface targets than if a light source were used alone (thus enhancing epidermal preservation).
1.LTIs are usually based on varying degrees of light absorption by tissue HgB, melanin, and water. 2.Wavelength ranges should be chosen to achieve as much specificity as possible in tissue heating. |
There are four key components in the sequence of most photothermal laser-tissue interactions (A–D).
(A) Beam Propagation: How the Laser Energy Gets to the Target
Skin optical properties determine the penetration, absorption, and internal dosimetry of laser light. The laser surgeon can divide the skin into two components, (1) the epidermis (primarily an absorber of visible light due to melanin) and (2) The dermis (which can be envisioned as a carton of milk with red dots in it). Light tissue interactions can be broken down into A. The transport of light in tissue, B. Absorption of light and heat generation in tissue, C. Localized temperature elevation in the target tissue (and denaturation of proteins), and D. Heat diffusion away from the target (Fig. 8).17,55
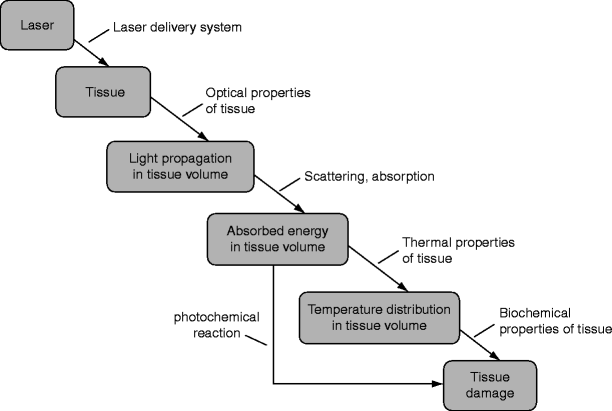
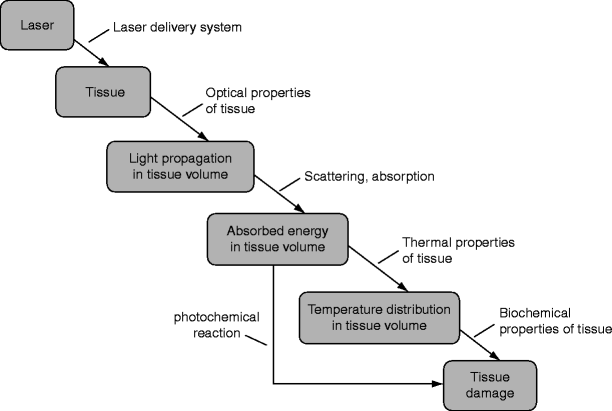
Fig. 8
Interaction between laser light and biological tissue and the resulting effects (From Knappe et al.18; Fig. 1)
The optical properties of the skin mimic a turbid medium intermixed with focal discrete visible and infrared light absorbers (blood, melanin, bilirubin, and dry collagen).56 The thermal or photochemical effects depend on the local energy density at the target. Once the light penetrates the surface, it undergoes a series of absorbing and scattering events. Photons statistically are either scattered or absorbed in a wavelength dependent fashion.1,57 Scattering is affected by the shape or size of the particle and the index of refraction mismatch between the particle and medium. For most tissues, for λ > 2.5 μm or less than 250 nm, absorption dominates over scattering. For the remainder of the EM spectrum, scattering is the primary attenuator of light in tissue with the exception of focal discrete absorbers (melanosome, HgB, etc.)
The probabilities of absorption or scattering (designated μa and μs respectively, Table 1) are determined by experiment. For example, for a μa of 0.3 cm−1, the mean free path before absorption is 1/μa or 3.3 cm. Generally, light is attenuated as it propagates through tissue. In turbid tissue (i.e., the dermis, where collagen acts as the major scatterer), the fluence attenuation can be described by:

where I(z) is the local subsurface fluence at some depth z, k is a constant that accounts for backscattered light and δ is the wavelength dependent optical penetration depth of light, or the depth at which there is attenuation to 37% of the surface value (37% = l/e, where e = 2.7, the base of the natural logarithm). This depth is determined by absorption and scattering coefficients, as related by the simple equation below1,57:
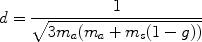

(3)
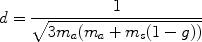
(4)
Where g is the anisotropy coefficient (a measure of the “mean” direction of the scattered photons). g = 0.9 for the skin. As μa and μs increase, δ decreases accordingly. For example, for hair removal, based solely on depth of penetration, longer wavelengths such as 800 and 1,064 nm should be preferable to 694 and 755 nm. In the visible light range, this is why red light can penetrate one’s hand when shining a flash light on the surface. Scattering decreases roughly proportional to λ3/2, so that, for example, an 800-nm photon will on average travel about 1.3 times as far in tissue as a 700-nm photon without being scattered. It follows that for “more” scattering wavelengths, there will be greater accumulation of photons near the surface. In addition to scattering, this superficial convergence of photons is based on index of refraction mismatches between air and tissue.1 Accordingly, light must be deposited more slowly with shorter wavelengths to avoid overheating the superficial tissue.
There is backscattered light that can yield a higher fluence beneath the tissue than at the tissue surface.58 This paradox of tissue optics is that the internal fluence can actually exceed that at the surface, as below:


(5)
Where I is the subsurface energy density, Io is the surface fluence, R = the surface remittance (0.3, 0.6, and 0.7 for 585, 694, and 1,064 nm respectively). (personal communication from RR Anderson, 1994)
Since neither macromolecules nor water strongly absorb in the red light and near IR (600–1,200 nm) this range allows deeper penetration in most tissues.10 Various skin pigments can play optical “tricks” on the cutaneous surgeon. For example, poikiloderma appears to be a mix of hyperpigmentation and hypervascularity. In fact, although there is some melanin influence in the red-brown appearance, the dyschromia is by far more a disorder of matted telangiectasia. This is confirmed by the good response of the condition to the PDL, even with aggressive surface cooling that should preclude any impact on superficial cutaneous hyperpigmentation. Additionally, with diascopy, “poikilodermatous” skin often appears no browner than the surrounding apparently normal skin. The explanation is that deoxy-Hb contributes to a “pigmented skin appearance”. This finding follows from the absorption spectrum of deoxy-Hb in the 630–700 nm range, which is very similar to the absorption spectrum of epidermal melanin. The size of the vessels in the superficial venous plexus is such that the transmitted light through these vessels is approximately 50% lower than the incident intensity. These vessels therefore appear dark.59
In most biological systems, tissue constituents show broad absorption bands with only a few distinct absorption peaks. From 200 to 290 nm (UVC), all biological objects (cells and tissue) absorb energy very strongly. From 290 to 320 (UVB) nm, only a limited number of biomolecules show absorption (aromatic amino acids and nucleic acids). For UVA 320–400 nm, light is weakly absorbed by colorless skin parts. From 400 to 1,000 nm mainly pigments- bilirubin, blood, and melanin absorb light. The heterogeneity of the skin allows for discrete heating over this range, and therefore selective photothermolysis (SPT) is exploited in this band. For >1,100 nm, all biomolecules have specific strong vibrational absorption bands. Tissue water is the primary determiner of the response to laser in this wavelength range.9
The absorption coefficient (μa) is the relative “probability” per unit path length that a photon at a particular wavelength will be absorbed. It is therefore measured in units of 1/distance and is typically designated μa, given as cm−1. The absorption coefficient is chromophore and wavelength dependent. For larger heterogeneous volumes, μa can be weighted according to the fraction of a specific chromophore. For example, for a dermis a typical blood fraction (f.blood) is 0.2%, assuming that the blood is uniformly distributed in the skin.7
Following the descriptive convention of describing an equivalent average homogeneous f.blood, the net absorption of the dermis, μa.derm, is calculated:


(6)
Scattering is responsible for much of light’s behavior in skin (beam dispersion, spot size effects, etc.). The dermis appears white because of light scatter. The main scattering wavelengths (relative to absorption) are between 400 and 1,200 nm. Absorption occurs where the laser frequency equals the natural frequency of the free vibrations of the particles (absorption is associated with resonance).60 Scattering occurs at frequencies not corresponding to those natural frequencies of particles. Scattering is decreased as wavelength increases.7
There are four major chromophores (water, blood, tattoo ink, and melanin) in cutaneous laser medicine.60 Water makes up about 65% of the dermis and lower epidermis. There is some water absorption in the UV. Between 400 and 800 nm, water absorption is quite small (which is consistent with our real world experience that light propagates quite readily through a glass of water). Beyond 800 nm, there is a small peak at 980 nm, followed by larger peaks at 1,480 and 10,600 nm. The water absorption maximum is 2,940 nm (erbium YAG).
Hemoglobin: There is a large HgBO2 (oxyhemoglobin) peak at 415 nm, followed by smaller peaks at 540 and 577 nm. An even smaller peak is at 940 nm. For deoxy-hemoglobin (HgB), the peaks are at 430 and 555 nm. The discrete peaks of hemoglobin absorption allow for selective vessel heating. Although the 410 nm peak achieves the greatest theoretical vascular to pigment damage ratio among the other peaks, scattering is too strong for violet light to be a viable option for vascular applications.
Melanin: Most pigmented lesions result from excessive melanin in the epidermis. By choosing almost any wavelength (<800 nm), one can preferentially heat epidermal melanin. Shorter wavelengths will create very high superficial epidermal temperatures, whereas longer wavelengths tend to bypass epidermal melanin (i.e., 1,064 nm).
Fat: Fat shows strong absorption at 1,200 and 1,700 nm.61 Although the ratios of fat to water absorption are small, the small differences are exploited with the proper choice of parameters. 1,200 nm might represent the best choice due to decreased overall water absorption and therefore increased penetration. Sebum is similar to fat but also is comprised of wax esters and squalene.
Carbon: Carbon is a product of prolonged skin heating. Once carbon is formed at the skin surface, the skin becomes “opaque” to most laser wavelengths (that is, most energy will be absorbed very superficially). It follows that the dynamics of surface heating changes immediately once carbon is formed. This can be used creatively as an advantage. For example, one can convert a deeply penetrating laser to one that would only affect the surface by using a carbon dye. This has been accomplished with a laser peel using a Q Switched Nd YAG laser.
Collagen: Dry collagen has absorption peaks near 6 and 7 μm. With a free electron laser operating at these wavelengths, collagen can be directly heated. Ellis et al. found that this approach might allow for less tissue irradiation and less thermal damage than CO2 laser.62
(B) Heat Generation
Selective Photothermolysis (SPT)
Non-bulk skin heating is based on selective absorption by discrete chromophores of relatively low concentration (i.e., melanin, hemoglobin). Dr. Leon Goldman showed that color contrast allowed for selective damage of dermal targets as early as 1963.63 However, it was Dr. RR Anderson who elegantly described the concept of selective photothermolysis.27 Selective photothermolysis offered a mathematically rigorous rationale for tissue-selective lasers. As described by Dr. Anderson, extreme localized heating
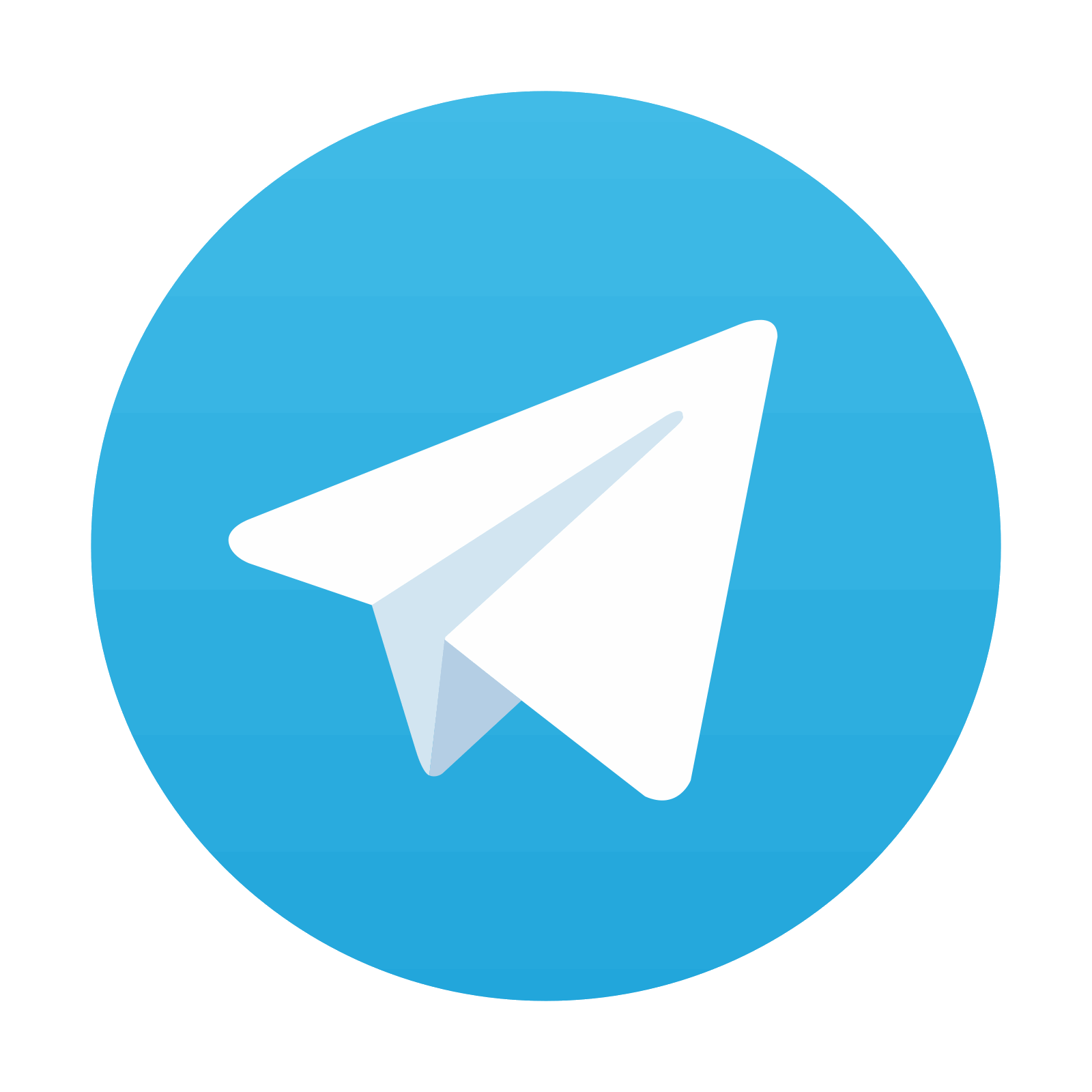
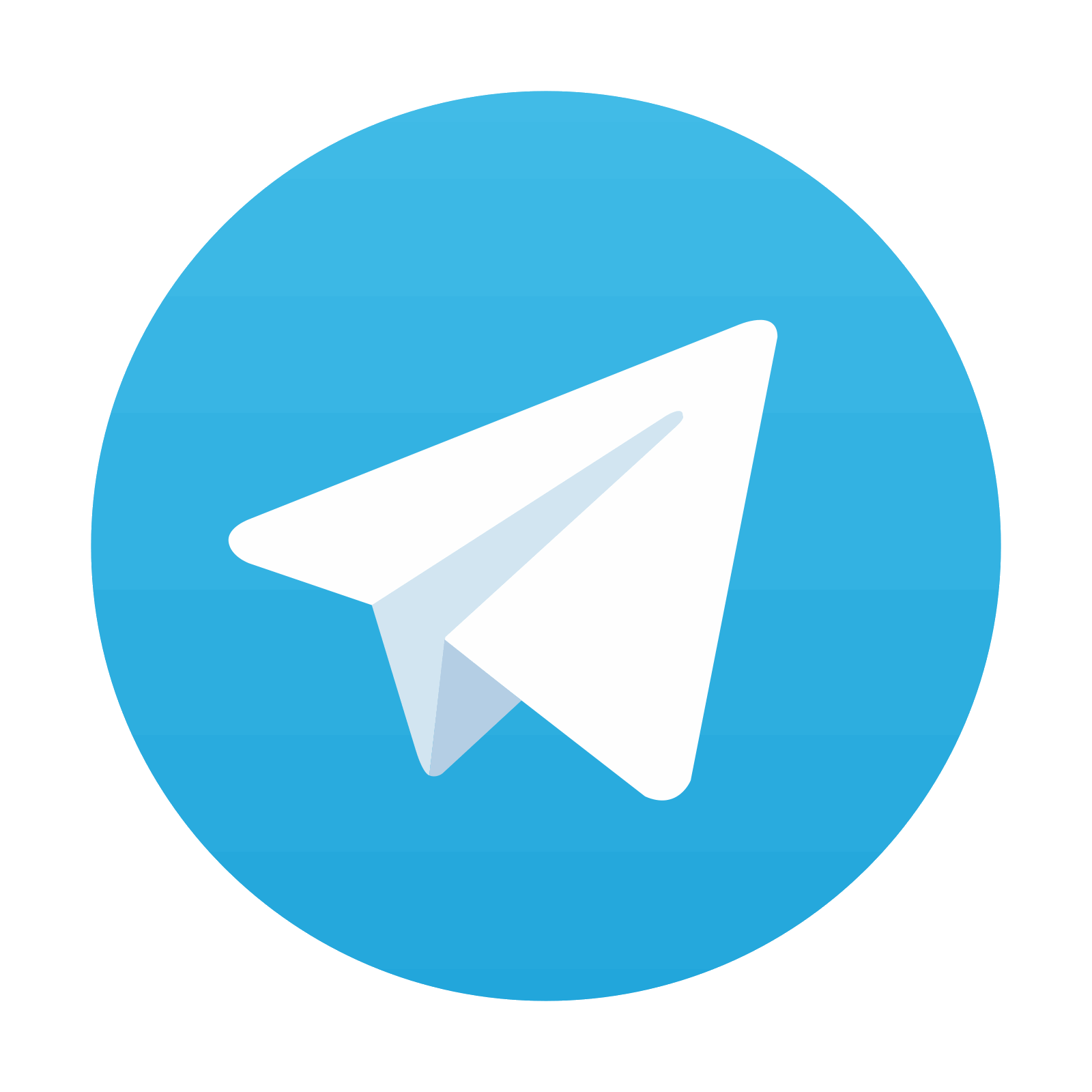
Stay updated, free articles. Join our Telegram channel
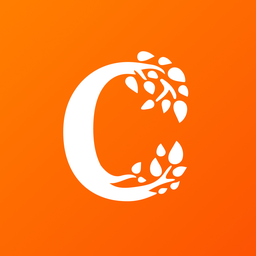
Full access? Get Clinical Tree
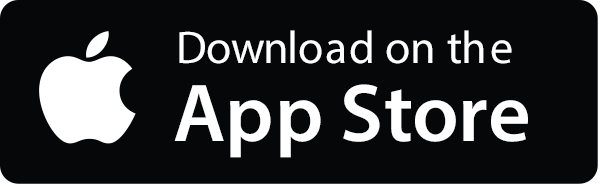
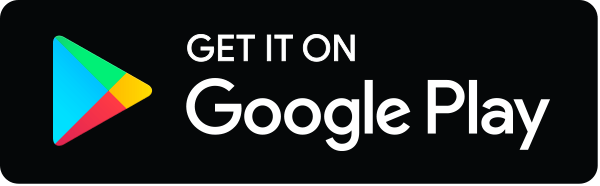