Fig. 7.1
In normal human skin (NHS), the SC may be subdivided into SC compactum, where the principal permeability barrier resides, and SC disjunctum, where the desquamation process takes place. Transition between the two regions is visualized in the inset. Several corneodesmosomes (small arrows) link SC compactum corneocytes both laterally and vertically. SC disjunctum is characterized by horizontal splitting between the successive corneocyte layers due to the loss of the interlayer corneodesmosomes. Exposure to propylene glycol (PG) induces basket-weave pattern in the entire SC. Interlayer corneodesmosomes become disrupted (arrowheads) because of a better accessibility of the catalytic enzymes, but the cells remain attached laterally (big arrows). This phenomenon is likely related to the presence of TJ-like structures sealing off the corneocyte periphery and therein located corneodesmosomes. Bars = 200 nm in NHS and 500 nm in PG
In transmission electron microscopy, TJ appear as fusion points between external sheets of the plasma membranes of two neighboring cells. Such cell–cell membrane fusions become cross-linked during the keratinization process and persist in the SC in a form of TJ-like structures (Haftek et al. 2011). The resulting subdivision of the intercellular spaces of the SC into independent compartments appears to be important for appropriate regulation of the desquamation process and, thus, the barrier homeostasis.
At the molecular level, TJ are composed of transmembrane and cytoplasmic proteins connected to the actin cytoskeleton (Brandner et al. 2002; Niessen 2007; Brandner et al. 2010). Several transmembrane TJ-specific proteins have been identified, e.g., occludin, several claudins, crumb, and JAM (junction adhesion molecules) (Fig. 7.2). Extracellular domains of these proteins interact to establish cell–cell contacts. The interaction between claudins engenders the formation of “pores” selective for various types and sizes of molecules (Tsukita and Furuse 2000; Furuse et al. 2002) (Fig. 7.3). The selectivity is dependent on the concentration and the type of claudins participating in the junction protein complex. Thus, the extracellular parts of TJ are not fully “tight.” However, when distributed as a continuous band around a cell, TJ efficiently subdivides the cell surface into the apical and basal regions thereby contributing to creation of cell polarity.



Fig. 7.2
Schematic representation of a tight junction (TJ) structure and of its cytoplasmic interacting proteins: transmembrane proteins, i.e., claudins and occludin, and junctional adhesion molecule (JAM) which interact in the intercellular space where linker proteins zonula occludens and zonula occludens-associated nucleic acid binding protein (ZO-1/3, ZONAB) attach TJ to the actin cytoskeleton. Regulatory proteins, e.g., Sec 6/8 the secretion complex, Pals linking protein, Patj Pals-linking protein to tight junctions, Par-3/6 partitioning defective 3 and 6, Crumb, Cdc42 cell division control protein 42, Rab, and Rho/Rac small G-proteins are important for TJ formation and signaling

Fig. 7.3
Schematic drawing of molecule-selective “pores” engendered by tight junction (TJ) proteins in the intercellular spaces between the interacting cells. Pore-like structures are responsible for the control of paracellular diffusion of different molecules through the junctions. Claudins control the “tightness” and the selectivity of the pores within the TJ strands and, thus, TJ function relays largely on their claudin composition. A 3D representation of the arrangement of TJ proteins at the cell surface as well as the “pores” created after interaction of the extracellular domains of claudins and occludin are shown to the left (a). To the right (b) – distribution of the lined-up transmembrane proteins as it can be visualized at the cell surface with freeze-fracture
Ken Hashimoto has observed TJ structures at the apical membrane of the SG keratinocytes as early as 1971 (Hashimoto 1971). However, this observation has been neglected for 30 years because lipids of the intercellular space of SC were considered the only efficient barrier to the water loss (Elias and Friend 1975). The beginning of the twenty-first century was marked by the revival of epidermal TJ studies. In 2002, Furuse et al. have demonstrated that biotin (557 Da) injected in a mouse dermis ascends to the epidermis via the intercellular spaces and stops in the SG at sites stained by antibody to occludin, thus indicating the presence of functional TJ at this level (Furuse et al. 2002). Moreover, in claudin-1 knockout mice that do not survive after birth due to the severe percutaneous water loss, the injected biotin crossed the level of SG, penetrating into the SC. This observation proved the presence of functional TJ in murine epidermis, capable of controlling the diffusion of molecules bigger than 557 Da.
In man, several authors have reported the presence of TJ-associated proteins in the epidermis and TJ-like structures in the SG of the fetal and, more recently, adult epidermis (Pummi et al. 2001; Brandner et al. 2002; Langbein et al. 2003; Schluter et al. 2004). These studies indicate that TJ provide a barrier against the permeability of solutes, also in human epidermis. However, doubt remains, concerning the exact role of TJ and their contribution to the barrier function, since the main permeability barrier at this anatomic site is apparently provided by the SC components.
One possibility is that TJ play an important role in case of rupture of the SC barrier and during the formation of this layer. In fact, TJ belts distributed around the granular layer keratinocytes could contribute to the apical extrusion of lamellar bodies. The oriented delivery of lipids to the interface between the viable epidermis and the SC is necessary for filling of the intercellular spaces with a hydrophobic material and thus for the correct barrier function (Brandner et al. 2010).
As cornified envelopes of corneocytes are impermeable to most diffusing substances, the main penetration pathway through the SC remains the intercellular one (Elias 1983). Lipids of the SC constitute the main component of the hydrophobic human skin barrier regulating water homoeostasis. Lipid composition determines the lipid organization in the SC and is therefore a key factor underlying the skin barrier function (De Jager et al. 2004). The lipid matrix is mainly composed of equimolar mixture of ceramides (CER, approx. 50 % by weight), free fatty acids (FFA; approx. 10 % by weight), and cholesterol (approx. 20 % by weight and its derivate cholesterol sulfate – approx. 5 %) (Wertz 2000; Weerheim and Ponec 2001; Feingold 2009). Triglycerides are also present in the SC barrier but, unlike viable cell membranes, the SC is almost completely deprived of phospholipids. Cholesterol plays an essential role in the maintenance of the membranes’ fluidity, as it promotes intermixing of different lipid species, whereas cholesterol sulfate takes part in the modulation of desquamation because of its inhibitory action upon serine proteases. FFA consists predominantly of saturated long-chain species. Oleic acid (6 %) and linoleic acid (2 %) are the only unsaturated fatty acids detected unbound in the SC.
To date, in human SC, 12 ceramide subclasses with a wide distribution of the chain length have been identified (Masukawa et al. 2009; Van Smeden et al. 2011). All ceramides bear a polar head group and two long carbon chains: a sphingoid base and a fatty acid. They are necessary for the formation of the covalently bound lipid envelope of corneocytes (Behne et al. 2000; Zheng et al. 2011) and play a key role in the functioning of the SC (Coderch et al. 2003). Ceramide-1 is particularly important for the intercellular lipid organization, because its long acyl chain is able to span more than one lipid bilayer and, therefore, it helps to rivet the multilayered matrix (Bouwstra et al. 2002). In general, a reduction in chain length of CERs has a stronger impact on the lamellar lipid organization and permeability than a change in the ratio between CER subclasses keeping the chain length approximately equal (De Jager et al. 2006; Groen et al. 2011; Neto et al. 2011). In atopic dermatitis patients, a reduction in overall ceramide chain length is observed and correlates with the impaired barrier function (Di Nardo et al. 1998; Janssens et al. 2012).
In normal human SC, lipids are arranged in two coexisting lamellar phases, a long periodicity phase (LPP) and a short periodicity phase (SPP) with repeat distances of around 13 and 6 nm, respectively (Madison et al. 1987; Bouwstra et al. 1991). Within the lipid lamellae, lipid head groups display an orthorhombic lateral organization (solid crystalline phase) at the skin temperature of around 30–32 °C. However, a subpopulation of lipids can also be less densely packed, showing a hexagonal pattern (gel crystalline phase) or, the most fluid, liquid crystalline phase (Bommannan et al. 1990; Ongpipattanakul et al. 1994; Pilgram et al. 2001a). Although the orthorhombic packing is predominant in the SC, the uppermost SC layers have the highest extent of the liquid crystalline phase, a consequence of the presence of sebum. As shown in in vitro experiments, cholesterol and ceramides are very important for the formation of the lamellar phases. After addition of FFA, the lipids are organized in an orthorhombic packing with a small proportion of lipids in a liquid crystalline phase. Deficiencies in any one of the three main lipid species result in barrier abnormalities as well as observable alterations in the ultrastructural features of the SC extracellular domains (Holleran et al. 2006). Lamellar lipid organization is considered to play an important role in the barrier function of the skin, especially the LPP (Bouwstra and Ponec 2006). Orthorhombic phase is considered as the least permeable structure, whereas the liquid crystalline phase is highly permeable to compounds (Groen et al. 2011). On the other hand, liquid phase seems to be necessary for formation of the LPP pattern (Bouwstra et al. 1999). Moreover, in dry skin, a pure liquid crystal system allows a rapid water loss through the bilayers with a moderate barrier action. The solid system could cause a water loss due to breaks in the solid crystalline phase (Fluhr et al. 2008). Maintaining the balance between the phases of different physical properties is probably required for optimal barrier function in preventing water loss (Thau 2002).
7.2 Excipients: Their Nature, Roles, and Modes of Action
According to the European Medicines Agency (EMA, earlier EMEA), excipients may be defined as the constituents of a pharmaceutical formulation which are not the active substance. Excipients include, e.g., coloring matters, antioxidants, preservatives, adjuvants, stabilizers, thickeners, emulsifiers, solubilizers, penetration enhancers, flavoring and aromatic substances, as well as constituents of the outer covering of the medicinal products.
Penetration enhancers are excipients which have the ability to modify the penetration of active substances through the skin and therefore could significantly influence the in vivo performance of a dermal and transdermal formulation. Development and control of action of these substances are essential for all dermal and especially transdermal formulations, where a constant and persistent release of active molecules over several hours or even days is mandatory for therapeutic efficacy.
7.2.1 Excipients’ Action on the SC Lipids
Most of the skin penetration enhancers are designed to modify the intercellular SC domains in order to reduce the resistance of barrier lipid bilayers. They affect the strength of interactions between the polar head groups, the conformational state, and/or the lateral packing of the lipids (Tfayli et al. 2012). Recent studies have also emphasized the effects of detergents on lipid synthesis, on lipid-metabolizing enzymes and, generally, on keratinocyte differentiation (Wei et al. 2006; Torma and Berne 2009). Several mechanisms of action are individualized and may intervene separately, depending on the nature of the excipient, although combined actions are most frequently encountered.
7.2.1.1 Extraction of Lipid Components
Ethanol at concentrations between 40 and 80 % extracts lipids from the skin and promotes the permeation of polar and nonpolar drugs through a hypothetical “pore” pathway (Manabe et al. 1996; Levang et al. 1999). Such lipid depletion or “degreasing” of the skin, i.e., the ability of solvents or detergents to solubilize and remove SC lipids, may also be involved in the damaging effect observed after prolonged or repetitive exposure to such substances.
7.2.1.2 Fluidization of the Intercellular Lipid Matrix
The enhanced permeation observed in the presence of ethanol was proposed to be associated with different mechanisms including the fluidization of SC lipids (Panchagnula et al. 2001). Fourier transform infrared (FTIR) experiment showed increase in SC lipid fluidity upon application of 20–60 % ethanol, which may enhance the permeation of lipophilic drugs, especially through the intercellular lipid pathway (Manabe et al. 1996).
7.2.1.3 Disorganization of the Lipid Lamellae
In normal SC, it is thought that the ratio of lipids in ordered and disordered (liquid crystalline) phases modulates the SC barrier function properties (Boncheva et al. 2008). The orthorhombic packing is the most tightly packed lipid barrier. It depends on the presence of long-chain fatty acids combined with ceramides in association with cholesterol (Bouwstra et al. 1998). Towards the skin surface, sebum lipids can induce a transition to a less tightly packed hexagonal phase (Pilgram et al. 2001b). Soaps associated with an extensive hydration, e.g., during bathing, can also promote a less organized state of the SC lipids (Rawlings et al. 1994). Moreover, some detergents induce horizontal splitting in the lipid layers leading to the loosening of the intercorneocyte spaces and thus facilitate the mobility of the extracellular enzymes implicated in the SC barrier modulation (Haftek et al. 1998) (Fig. 7.4; see also Fig. 7.1).


Fig. 7.4
Ultrastructure of human stratum corneum compactum post-fixed with ruthenium tetroxide (RuO4): (a) nonoccluded; (b, c) simple occlusion; (d–g) permeabilization with propylene glycol. Electron-dense lenticular dilatations (arrowheads) are localized between the intercellular lipid lamellae. In the occluded SC, these hydrophilic compartments are often found in contact with corneodesmosomes (open arrows). Propylene glycol promotes further expansion of the dark pools of intercellular substance and corneodesmosome degradation. The junctional plugs appear frequently fragmented and unevenly stained. At places where the corneodesmosome edges remain in direct contact with equally electron dense dilations, distinction between the two elements is difficult (f, g). Typical triple-band structures of the corneodesmosome cores are observable mostly with low levels of RuO4 staining, rendering lipid lamellae less visible (Reproduced with permission from Microscopy Research and Techniques, John Wiley and Sons; Haftek et al. (1998))
7.2.2 Excipients’ Action on the Tight Junctions
TJ modulators interact with the extracellular loops or the membrane domain of TJ. Such modulators or excipients can belong to various families of molecules, i.e., lipids, surfactants, and proteins (Wong and Gumbiner 1997; Kondoh and Yagi 2007; Kondoh et al. 2008; Johnson et al. 2008). Wong et al. have proved that a 44-amino-acid-long peptide is able to increase the cellular turnover of occludin, one of the TJ complex proteins (Wong and Gumbiner 1997). This reversible effect results in an increase of the paracellular permeability of TJ.
Another example of a peptide capable of disintegrating the TJ structure by simple interaction with one of its proteins is the C-terminal fragment of Clostridium perfringens enterotoxin. This peptide interacts with claudin-4 and leads to the internalization of TJ. It could be used as a poison that provokes the complete loss of the intestinal TJ barrier (Morita et al. 1999; Sonoda et al. 1999).
7.3 Methods to Evaluate Influence of Excipients on the Epidermal Barrier
7.3.1 Methods to Follow the Structural Modifications
7.3.1.1 Microscopy Methods
Microscopy can be used to detect the influence of excipients on the extracellular lipid matrix as well as on the TJ. Light microscopy combined with immunodetection is usually employed to follow changes in expression and distribution of protein components such as TJ molecules. Immunofluorescent studies performed on frozen tissue sections allow the evaluation of “native” tissue, not fixed chemically and not exposed to solvents during the preparative methods. When combined with exploration in confocal laser scanning microscopy, where virtual optical sections are recorded within the immunolabeled tissue, this approach is very useful to evaluate TJ topography in 3D reconstructions (Brandner et al. 2002; Kubo et al. 2009). Immunostaining of dewaxed skin sections, following the standard histology protocol involving aldehyde fixation and paraffin embedding, should be interpreted with care when the SC is the compartment of interest. Indeed, solvents used during tissue dehydration and then during dewaxing efficiently remove intercorneocyte lipid matrix thus rendering impossible observation of excipient-induced changes in this localization. On the other hand, lipid removal may be beneficial for studies of TJ antigens persisting in the SC, as it improves accessibility of the latter to adequate antibodies.
Electron microscopy approach gives further insight into the structural composition of the SC barrier elements. Its “standard” transmission electron microscopy version, consisting of aldehyde fixation followed by osmium tetroxide post-fixation and resin embedding, is less suitable for studies of the SC barrier, essentially for the same reasons as the “standard” light microscopy. Although osmium tetroxide is able to partially preserve lipid bilayers from extraction during the subsequent dehydration procedures, visualization of the fine lamellar structures, within the extracellular spaces of SC, is not possible with this technique. Ruthenium tetroxide post-fixation solves this problem; however, incubation with this highly oxidizing reagent results in significant deterioration of the ultrastructure of living cells (Swartzendruber et al. 1995). Low-temperature embedding of samples in partially hydrophilic acrylate resins also preserves, to some extent, lipids from extraction by solvents used during the dehydration phase. However, it is not compatible with osmium or ruthenium tetroxide post-fixation because polymerization takes place under ultraviolet radiation which poorly penetrates tissue blocks turned black by the fixatives (Haftek et al. 1998). Acrylate-embedded samples are widely used for on-section immunolabeling with colloidal gold, including that of TJ (Haftek et al. 2011). Efficiency of ultrastructural immunolabeling can be further improved by the use of non-resin-embedded tissue ultra-cryosections (Igawa et al. 2011). Transmission electron microscopy is also used for observation of freeze-fracture samples, with or without immunolabeling (Corcuff et al. 2002). In this approach, the snap-frozen tissue is broken into pieces and the resulting fracture profile is covered with metal atoms. After removal of the underlying tissue, the metallic replica is examined with an electron microscope. Exposed fragments of the plasma membranes, where the split occurs preferentially, may show the presence of transmembrane molecules, such as TJ proteins (Kurasawa et al. 2009). The latter are arranged in a characteristic crisscrossed linear pattern and their TJ origin may be confirmed using immunogold labeling of the replicas with specific antibodies. Also, freeze-fracture studies efficiently help to investigate the lipid arrangement within the SC extracellular spaces (Van Hal et al. 1996).
Scanning electron microscopy methods appear to be less suited for SC barrier studies because of the magnification range, which is much lower than in the transmission variant, and due to accessibility limited to the surface of a sample. Nevertheless, recent technological improvements allowing examination in partial vacuum with signals obtained with secondary and retro-diffused electrons herald new applications using tape-stripped and immunolabeled SC [Haftek 2013, unpublished data].
7.3.1.2 Vibrational Spectroscopies
Vibrational spectroscopies are powerful nondestructive techniques that detect characteristic vibrational energy levels of a molecule. Infrared and Raman spectra contain detailed information about the structures and interactions of molecular classes of interest. Several studies have reported the potential of FTIR and Raman spectroscopies for the characterization of the molecular and supramolecular organization of lipids and to follow changes in the lipid chain conformational order. More particularly, these techniques have already been used to characterize phase transitions of the SC lipids (Moore et al. 1997; Lawson et al. 1998; Ponec et al. 2000; Wartewig and Neubert 2007) of related model systems (Bouwstra et al. 2002) and to study polar and nonpolar lipid–lipid interactions (Corbe et al. 2007).
7.3.1.3 FTIR: Fourier Transform Infrared Spectroscopy
FTIR was used to obtain information on the lateral lipid organization and conformational ordering of the lipids (Janssens et al. 2012). FTIR spectroscopy can be used to investigate the biophysical alterations taking place in the lipid bilayer after treatment with penetration enhancers by studying the vibrational modes of its components. Different types of vibrations were monitored, the CH2 symmetric stretching vibration and second derivatives of the scissoring bandwidth being the most informative for lateral organization (Boncheva et al. 2008; Damien and Boncheva 2010). For instance, the CH2 symmetric and asymmetric stretching frequencies (υsym CH2 and υasym CH2) near 2,850 and 2,920 cm−1, respectively, are primarily sensitive to lipid chain conformational order. A low (~2,848 cm−1) wave number of the CH2 symmetric stretching vibrations indicates the presence of a highly ordered lipid organization (either hexagonal or orthorhombic), while a high (2,853 cm−1) wave number indicates the disordered liquid phase (Moore et al. 1997). However, a low average bandwidth of the scissoring vibrations represents a reduction of lipids in an orthorhombic organization and thus a less dense lipid organization (Janssens et al. 2012). Extraction of the SC lipids with solvents results in reduction of the CH2 stretching absorbance. Indeed, the decrease in CH2 stretching bandwidths accompanied by a decrease in CH2 stretching band intensity suggests an overall extraction of SC lipids (Levang et al. 1999). The CH2 rocking frequencies (710–735 cm−1) are much more sensitive than the changes in CH2 symmetric frequencies to the aforementioned hexagonal–orthorhombic phase transition. At temperatures below 40 °C, there are two distinct bands at approximately 720 and 729 cm−1. The latter is a reliable marker of an orthorhombic phase in the SC lipids (Pensack et al. 2006).
7.3.1.4 Differential Scanning Calorimetry (DSC)
DSC is a thermoanalytical technique measuring heat capacity of a sample. It allows thermal analysis of an isolated SC and is used to evaluate the interaction of excipients with the skin.
Alterations of the characteristic temperatures of the endothermic peaks can be observed, relative to disorganization of the lamellar structure of lipids and to dehydration or denaturation of the proteins. DSC is less sensitive to phase transitions, presumably because of relatively low enthalpy changes resulting from the transition, whereas FTIR is able to detect them with relative ease.
7.3.1.5 Raman Spectroscopy
Raman spectroscopy reveals the spectral features specific to the modes of action of the penetration enhancers. These features can be simultaneously used as direct vibrational descriptors of the supramolecular organization, the polar interactions, and the conformational order of the CERs and as a descriptor of the penetration enhancer activity in drug delivery. Raman spectroscopy can be used to monitor not only the evolution of the lateral packing and the polar interactions due to penetration enhancer activity but also the intra-chain conformational order and the chain-end conformers, such as resulting from the thermotropic behavior of ceramides (Tfayli et al. 2010). Such information cannot be obtained with FTIR spectroscopy.
7.3.1.6 X-ray Diffraction Studies
In this method, a primary X-ray beam, emitted by a source, is partially scattered by the structures present in a studied sample. When applied to SC, the obtained diffraction patterns give information about organization of the intercellular lipids. Position and intensity of the observed peaks are measured and interpreted, in order to evaluate the action of excipient on lipid organization (Bouwstra and Ponec 2006).
Small-Angle X-ray Diffraction (SAXD)
Diffraction pattern measured at low angle, typically between 0° and 5°, provides information about the larger structural units in the sample, such as the repeat distance of a lamellar phase (Janssens et al. 2012).
At room temperature, two lamellar phases are present in human SC. One shows a periodicity of approximately 6.4 nm and the other of approximately 13.4 nm (Bouwstra et al. 1991). Since the 13 nm lamellar phase has always been present in all species studied so far, this phase is considered to be important for the skin barrier function.
Wide-Angle X-ray Diffraction (WAXD)
At wider angle, scattered X-rays contain information about smaller structural units in the sample, such as the lateral packing of molecules in a lamellar phase. In the liquid crystalline packing, the intermolecular distance is not very well defined. This results in a WAXD pattern that is characterized by a very broad peak at 0.46 nm. The diffraction pattern of the two-dimensional hexagonal lattice is characterized by a strong reflection at 0.41 nm. In the case of the orthorhombic organization, two strong reflections can be detected at a spacing of 0.41 and 0.37 nm. However, whether a hexagonal sublattice coexists with an orthorhombic one cannot be deduced from the diffraction pattern, since the 0.41 nm reflection, characteristic for the hexagonal lateral packing, is obscured by the 0.41 nm reflection attributed to the orthorhombic phase (Bouwstra and Ponec 2006).
7.3.2 Methods to Follow Biochemical Modifications
The presence of proteins of the TJ complex or enzymes implicated in the synthesis/degradation of lipids can be studied in tissue extracts. Similarly, quantitation of the corresponding messenger ribonucleic acid (mRNA) is also possible. As initial tissue localization of the obtained profiles is essential, these studies require precise sampling based on the structural data.
7.3.2.1 Molecular Biology
Molecular biology approach is required for the comprehension of viable cell responses. As such, it concerns exclusively studies of the living epidermal layers and not the SC. However, study of RNA gives an idea on the transcription rate of a specific gene and this, in turn, indicates possible repercussions on the subsequent SC formation and function. For example, quantification of mRNA coding for TJ proteins is possible using real-time polymerase chain reaction (RT PCR). Using this technique, one can monitor the presence of mRNA of various TJ proteins in keratinocyte cultures with different knockdown genes while testing permeability of the expressed TJ (Kirschner et al. 2013). Already in 2008, Yamamoto et al. have performed a posttranscriptional gene silencing of different TJ proteins to study the impact of these proteins on the formation of the SC barrier (Yamamoto et al. 2008). Keratinocytes were transfected with small interferent RNA (si-RNA) targeting either claudin-1 mRNA or occludin mRNA. It turns out that transfected cultures were not able to develop a functional barrier. Using molecular biology techniques, it would be possible to detect variations of the keratinocyte gene activity that excipients may engender when applied on the skin. Hybridization in situ, on tissue sections, is an approach combining structural information with mRNA detection. It is, thus, particularly well suited for studies of locally induced changes following topical treatments.
7.3.2.2 Biochemistry
Biochemistry assays are commonly used to analyze proteins as well as lipids extracted from a tissue.
Immunoblotting technique can detect the presence of a given protein. In fact, the presence of the mRNA in the cell cytoplasm is insufficient to predict the presence of the corresponding protein due to the complexity of the translation machinery. Western blot is a reliable qualitative and quantitative technique applicable to TJ protein studies (Kurasawa et al. 2009; Kirschner et al. 2013).
Lipid analysis may be performed on SC extracts using high performance thin layer chromatography (HPTLC) (Rissmann et al. 2008; Popa et al. 2012). Combined with sequential tape-stripping, HPTLC approach allows following lipid composition of intercellular spaces between corneocytes in the SC according to the sample’s depth (Popa et al. 2011).
7.3.3 Methods to Assess the Permeability Barrier Function
7.3.3.1 Transepidermal Water Loss (TEWL) Measurement
One of the key parameters to monitor the skin barrier function is the transepidermal water loss, i.e., the rate of water evaporation through the epidermis, independent of sweating. TEWL provides a noninvasive method for assessing changes in the barrier properties of the SC. It can be considered as a determinant indicative of the functional state of the epidermal barrier in normal, pathological, and experimental conditions (Janssens et al. 2012). Measurements are performed in standardized ambient conditions according to the well-defined consensus guidelines (Pinnagoda et al. 1990).
7.3.3.2 Diffusion Cells: Ex Vivo Permeation Studies
Diffusion cell is helpful to study the influence of excipients on drug permeation and penetration. Indirectly, information is gained on the functional state of the treated skin barrier. Skin permeation is evaluated by measuring the steady-state permeation flux of the tested drug. An enhancement ratio can be determined in the presence of the studied excipient. The diffusion cell consists of the upper, i.e., “donor,” and the lower, i.e., “receptor,” chambers, separated by a tested skin. The epidermis faces the donor chamber where the tested products are applied, and the dermis faces the receptor chamber filled with a receptor fluid, where the drug or another tracer will be measured after permeation. Temperature is controlled throughout the experiment and should be maintained at in vivo skin conditions (32 °C). Ensuring that the skin used for testing maintains its physical integrity is an essential factor to the successful performance of permeation experiments, as specified in the test guideline OECD 428 (Lévêque et al. 1993). The guidance document recommends the measurement of TEWL, transepithelial electrical resistance, or the use of tritiated water as permeation markers.
7.3.3.3 Transepithelial Electrical Resistance (TEER)
TEER measurement can be used to detect the TJ functionality status. Usually the functionality of TJ is evaluated in vitro on monolayer of cultured cells or on reconstructed human epidermis. Functional TJ significantly increase the tissue impedance towards a very weak electrical current generated by ohmmeters, by virtue of limiting ion flux through the intercellular space (Kurasawa et al. 2009; Abdayem et al. 2011). In normal human skin, SC by itself is able to prevent the passage of the testing current. Therefore, to detect any variation of resistance due to TJ modulation, the SC has to be partially or completely removed. This is usually done by tape stripping. In the case of in vitro reconstructed epidermis, the extracellular lipid matrix is not perfectly organized (Ponec et al. 2000) and the SC is fully hydrated. This results in readable TEER measurements, as the electrical current is able to cross this imperfect SC barrier (Abdayem et al. 2011). For the detection of the effects of different excipients on the TJ barrier, reconstructed epidermis is cultured in plastic inserts equipped with a permeable polycarbonate bottom. This experimental setting allows positioning of the ohmmeter electrodes on both sides of the cultured tissue. Tested excipients can be added at different doses either topically, i.e., directly on the SC surface, or “systemically,” into the feeding medium penetrating the reconstructed epidermis from the bottom compartment. In case of “systemic” treatment, excipients are in direct interaction with the epidermal TJ and the SC remains intact. Topically applied agents have first to cross the SC barrier, before acting on TJ situated in the granular layer. Thus, TEER readings in the latter case reflect both TJ and SC permeability modulation.
7.3.3.4 Dye Penetration Test
Intact SC and functional epidermal TJ are able to block or to limit the passage of various molecules depending on their size and nature, e.g., lipophilicity, pH, and charge. Following the passage of a tracer applied at the surface of the skin or injected at the basal layers of the epidermis is another way to study the functional quality of the complementary SC and TJ barriers. For example, in the case of topical application of Lucifer yellow, detection of this hydrophilic fluorescent dye, with a confocal microscope, in the living layers of epidermis, may indicate the presence of poor SC barrier, either constitutive or induced (Kurasawa et al. 2009). Another widely used small molecular marker is biotin (433–666 Da) (Ding et al. 2011). After being injected to the dermis, the tracer diffuses through the intercellular spaces until it reaches the TJ barrier in the stratum granulosum. Immunohistochemical detection of the biotin on vertical skin sections reveals the sites where the diffusion has been stopped. These points are recognized as TJ because of their co-localization with anti-occludin staining (Furuse et al. 2002). Tracer penetration studies can also be performed at the ultrastructural level using electron-dense lanthanum salts. Ken Hashimoto was the first to describe TJ structures in human epidermis using, precisely, this approach (Hashimoto 1971).
7.4 Known Effects of Excipients on the Epidermal Barrier Structure and Function
Excipients influencing skin barrier demonstrate properties of penetration enhancers and are employed accordingly. The modes of action of skin penetration enhancers involve, in general, either the disruption of skin barrier properties or the increase of drug partitioning into the various layers of the skin, starting with the SC.
7.4.1 Propylene Glycol (PG)
PG is a small organic and hydrophilic molecule widely used as solvent and excipient. It is considered nontoxic and shows low relative irritancy in vivo (Lashmar et al. 1989; Barry 1991; Haftek et al. 1996). Haftek et al. (1998) have evaluated the ultrastructural spatial organization of the intercorneocyte spaces before and after application of PG. When applied ex vivo undiluted on the skin, PG was capable of inducing a pronounced loosening of the horny layer, observable in light and electron microscopy as the “basket-weave pattern.” At the ultrastructural level, the SC dissociation could be linked to the expansion of water-containing domains within the intercorneocyte spaces associated with disorganization of the lamellar lipids and corneodesmosome degradation (Fig. 7.4). Thus, incorporation of PG into lipid layers does functionally change the properties of the intercorneocyte space and may increase mobility of the extracellular proteolytic enzymes (Haftek et al. 1996). This, in turn, promotes digestion of the intercorneocyte junctions and leads to desquamation. Interestingly, the PG-induced horizontal splitting occurring between the successive layers of corneocytes is not extended to the lateral cell–cell attachments (Fig. 7.1). Recent investigations on the TJ structures persisting in a cross-linked form between the SC corneocytes shed light on this curious phenomenon (Haftek et al. 2011). In fact, TJ-like structures located at the top of and within the lateral intercorneocyte contacts efficiently limit accessibility of the intercellular enzymes to the cell periphery and the corneodesmosomes located within it.
PG is also frequently employed to enhance drug release, from the dosage form (Panchagnula et al. 2001).
7.4.2 Fatty Acids: Oleic Acid (OA)
Percutaneous drug absorption is increased by a wide variety of long-chain fatty acids. Among the most frequently used is OA, as it moderately increases both drug diffusivity and drug partitioning parameters (Koyama et al. 1994). This long-chain monounsaturated fatty acid in cis configuration modifies the lipid domains of the SC. This effect appears to be due to lipid layers fluidization and, predominantly, to phase separation (Naik et al. 1995). Indeed, after in vivo application, OA has been found to exist in a liquid phase at all levels of the spectroscopically examined SC. OA can be applied on the skin in mixture with ethanol and easily combined with other penetration enhancers, like propylene glycol or terpenes (Yamane et al. 1995; Larrucea et al. 2001).
7.4.3 Terpenes
Terpenes are organic compounds found in essential oils. They are used as co-enhancers of percutaneous penetration, and, thus, their influence is measured as the difference when compared to the results obtained with control mixtures that do not contain them. Koyama et al. evaluated the percutaneous absorption-enhancing effects of d-limonene in oleic acid using three drug models with different lipophilicities (Koyama et al. 1994). Pretreatment of the skin with limonene resulted in a large penetration enhancement for lipophilic and amphiphilic molecules but had little effect on the hydrophilic one. D-limonene increased mainly drug diffusivity in the nonpolar penetration route. Moreover, carvone, 1,8-cineole, and thymol were shown to enhance the percutaneous absorption of hydrophilic 5-fluorouracil in 50 % ethanol by either increasing the SC lipid fluidity, as revealed with FTIR, or perturbing the barrier integrity of the epidermis, as demonstrated with TEWL (Gao and Singh 1997).
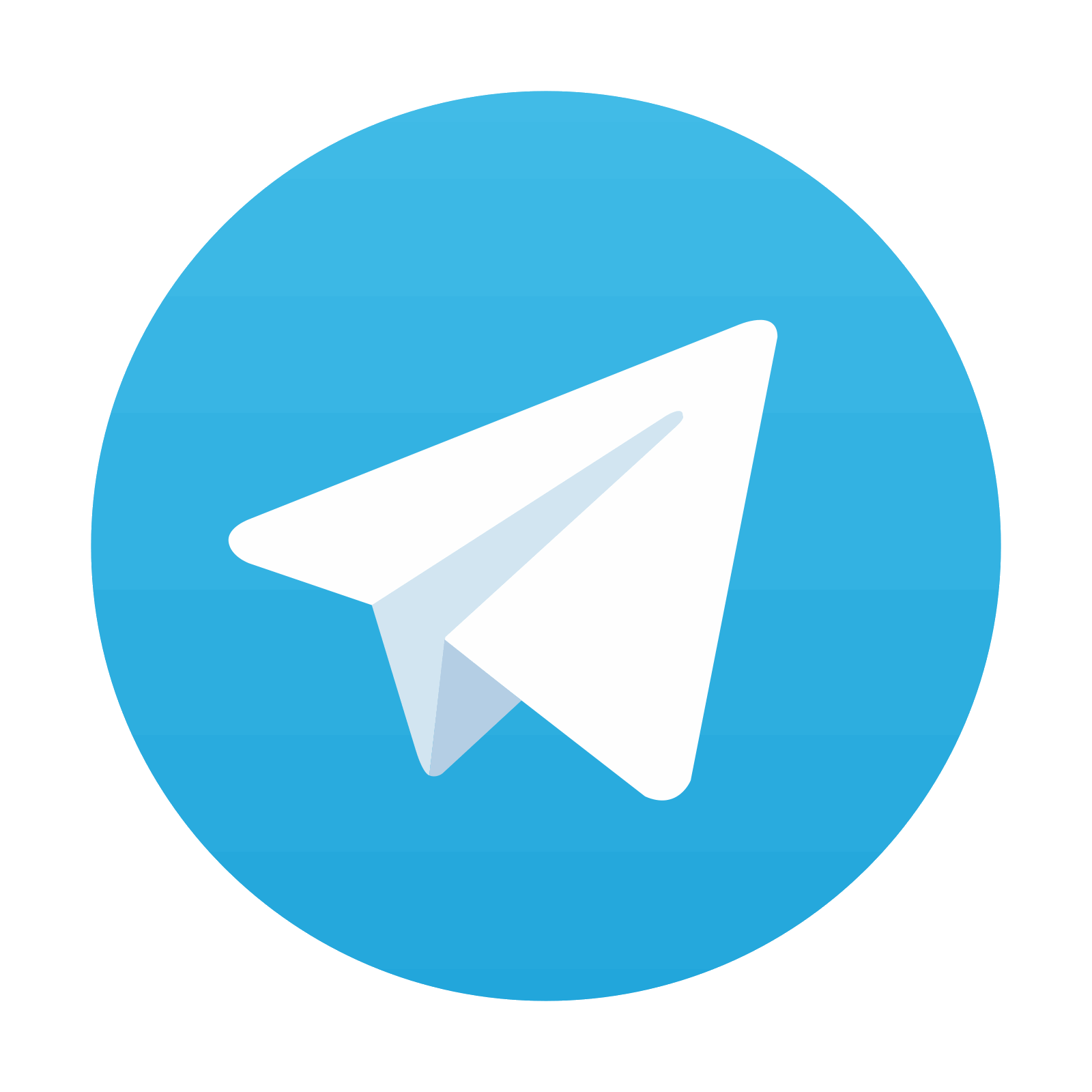
Stay updated, free articles. Join our Telegram channel
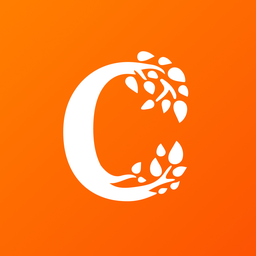
Full access? Get Clinical Tree
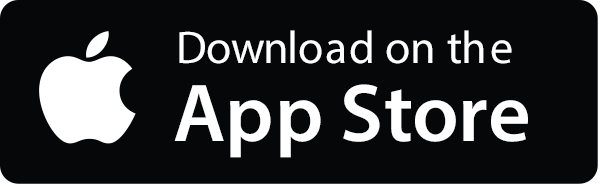
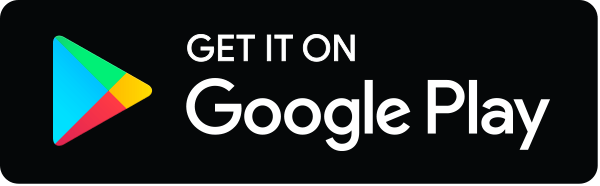