12 Hair Transplantation: The Promise of Cell Therapy
Summary
The next revolution in the field of hair transplantation will likely be brought about from the laboratory, where advances in cell culture, tissue engineering, and stem cell science have fueled promise of “lab-grown hair.” Technologies in bioengineered hair follicles and skin constructs will soon supplement hair transplantation, which up to now has been constrained by limited donor tissue. This chapter discusses our current understanding of the hair follicle and its cellular interactions, and the successes and aspirations of the basic science community in achieving these goals.
Keywords: tissue engineering stem cells induced pluripotent stem cells hair follicles skin constructs
Key Points
•Understanding the different classes of stem cells within the hair follicle, and how they interact with each other, and the scalp microenvironment, enlightens us on ways to reconstruct them ex vivo.
•Limited autologous donor tissue can be circumvented with stem cell technologies such as induced pluripotent stem cells, which allows a theoretically limitless supply of hair follicle stem cells for regenerative purposes.
•Tissue engineering techniques allow us to “rebuild” the skin and hair follicle with stem cell, and ultimately to generate hair follicles fit for transplantation.
12.1 Introduction
The value of a full head of hair has provided significant momentum to the technological progress seen in the field of hair transplantation. Incremental refinement of surgical techniques has seen the evolution of hair transplantation from scalp flaps to punch grafting of single follicular units (FUs; Fig. 12.1a). Harvesting of donor tissue, usually from the nonalopecic occipital scalp, has also become less invasive, such that each individual FU can be efficiently extracted. Despite these advances, donor FUs remain the limiting factor, as there is a finite, precious supply on the occipital scalp, which are relatively androgen insensitive compared to recipient frontoparietal regions. The next revolution in hair transplantation will likely stem from ex vivo techniques of expanding hair follicle stem cells (HFSCs) and using their regenerative potential for hair replacement (Fig. 12.1b).
Fig. 12.1 With advances in cell culture and tissue engineering, the problem of limited donor tissue (a) can be overcome by ex vivo expansion of hair follicle stem cells to create more follicles (b) for transplantation.
Each hair follicle (HF) is composed of several types of stem cells, in both the epithelial and mesenchymal compartments. These stem cells have varying levels of multipotency, and interact with each other to coordinate the development and subsequent cycling of the HF. These stem cells reside in specialized niches, which receive signals from neighboring cells, as well as extrafollicular cells, to provide the stem cells with instructions regarding self-renewal, quiescence, and proliferation/differentiation.1 Disruption of these niches has been hypothesized to lead to loss of stem cells, resulting in various forms of alopecia. For example, alopecia areata results from a breakdown in immune privilege that allows for an autoimmune attack on the hair bulb, where the dermal papilla and hair germ reside (Fig. 12.2). Androgenetic alopecia (AGA) is believed to be a result of multiple factors, including disrupted environmental signals in the form of androgens, which lead to the miniaturization of the HF from stem cell dysfunction and senescence.
Fig. 12.2 Hair follicle stem cells reside in the bulge region (red) and hair gem (green) of the hair follicle. Inductive signals arise from the dermal papilla (DP, cyan), which have stem cells in the dermal sheath (dark blue). Derm signals and microenvironmental cues contribute to regulation of hair cycling.
The main source of epithelial stem cells in the HF resides in the bulge (Fig. 12.2), which is situated close to the insertion of the arrector pili muscle. Bulge stem cells are long-lived, quiescent, and are able to differentiate into all lineages of the HF, and even the interfollicular epidermis when required (e.g., during wound healing). Bulge HFSCs give rise to a second population of stem cells known as the hair germ, which is more responsive to surrounding signals and proliferates and differentiates into the cells that produce the inner and outer root sheaths, companion layer, cuticle, and ultimately the hair shaft.
The most well-studied mesenchymal signals that coordinate the actions of the bulge and germ arise from the dermal papilla (DP),2 which is a specialized bud of mesenchymal cells at the base of the HF. The DP is absolutely required for anagen (growth phase) progression of the HF, and is the source of Wnts and fibroblast growth factors that promote proliferation and differentiation of the hair germ. As the hair cycles, the DP is replenished by stem cells that reside in the dermal sheath (Fig. 12.2).3
Extrafollicular signals also contribute to HF maintenance. This property is transferred together with the FUs during hair transplantation surgery. In addition, other growth factors present in the plasma are believed to provide support to transplanted FUs, providing evidence that immune cells or other migratory cells may produce factors and cytokines that regulate HFSCs. These must be considered in research efforts to recreate a sustainable microenvironment for HF regeneration using cell therapy.
12.2 Stem Cells for Hair Regeneration
In order to harness the regenerative potential of HFSCs, techniques to isolate, culture, and engineer new follicles have been developed to facilitate their manipulation.4,5
12.2.1 Primary Cell Therapy
Primary DP and HF stem cells have been used to establish cell lines. The hair-inducing DP can be isolated and expanded in vitro for cell therapy. Under monolayer culture conditions, however, DP cells lose their hair inductivity, which can be restored by culturing the cells under three-dimensional (3D) conditions.6 Nevertheless, the limited availability of primary cells, due to the limited replicative lifespan, often compromises the feasibility of primary cells for clinical applications. Primary cells have a finite lifespan, and telomere attritions caused by DNA replications eventually give rise to senescence (growth arrest). In addition, suboptimal in vitro culture conditions lead to oxidative stress in the cells, resulting in premature senescence before telomeres reach critical length. Senescence can be circumvented by immortalization (e.g., by genetic manipulation of the telomeres), but these alterations, along with mutations acquired from long-term passaging, make these types of primary cell lines unsuitable for use in patients.
The limitation on expansion of primary cells necessitates large-scale manufacture of allogeneic cell products to recruit multiple cell donors. The procurement of clinical-grade cells is a daunting task, because the cells need to be disease and viral free, which requires a strict and costly screening process. Additionally, donor variability compromises clinical applicability of the cells.
Embryonic stem cells (ESCs) do not senesce and have the potency to differentiate into desired cell types for cell therapy, but ethical issues are always a concern. Fortunately, induced pluripotent stem cells (iPSCs) have theoretically unlimited ESC-like capacity for cell therapy without the ethical concerns associated with ESC technology.
12.2.2 Induced Pluripotent Stem Cell Technology
Induced pluripotent stem cells are reprogrammed from primary adult somatic cells and exhibit a pluripotent stem cell–like state similar to ESCs.7 iPSCs do not exist in the human body, and are generated by forced expression of certain transcription factors, such as the four OSKM factors (Oct4, Sox2, Klf4, and c-Myc). Due to their unlimited proliferative potential and the potency to differentiate into many cell types, iPSCs can serve as an important new source of cells for drug discovery, cell therapy, and basic research. Moreover, iPSCs can be reprogrammed from easily accessible cell sources, circumventing the issues imposed by limited cell availability (Fig. 12.3).
Fig. 12.3 Schematic representation of the clinical pipeline to generate induced pluripotent stem cells from patient cells for regenerative purposes.
Sources of patient cells for iPSC generation are chosen based on their accessibility and reprogramming potential, which is variable in different somatic cell types. Keratinocytes have a higher reprogramming efficiency and can be reprogrammed more rapidly than skin fibroblasts under the same conditions using the four OSKM factors.8 Other somatic cell types may already express some of the four reprogramming factors at adequate levels, making them much more amenable to manipulation. For example, melanocytes express Sox2 endogenously at high levels, which makes Sox2 dispensable in the reprogramming cocktail.9 Intriguingly, DP cells only need the introduction of one additional factor (Oct4) for efficient reprogramming.10
Other considerations for implementation of a viable iPSC-patient service include the ability to store the cells for prolonged periods of time, as well as the accessibility of the somatic source cell types. Since the procurement of human fibroblasts, keratinocytes, or dermal papilla cells from skin or scalp biopsies requires a relatively invasive procedure, other noninvasive cell sources have been considered. It has been demonstrated that cells from urine samples and (cord) blood samples can be used for reprogramming.
Fibroblasts, keratinocytes, DP from the skin, and (peripheral blood mononuclear cells PBMCs) have all been successfully reprogrammed into iPSCs.11,12,13,14 These iPSCs can be redifferentiated into keratinocytes, fibroblasts, melanocytes, and endothelial cells using established protocols.14,15,16,17 iPSC-derived cells have been shown to interact and function as well as their primary counterparts. For example, melanin transfer to iPSC-derived melanocytes have been transferred successfully to iPSC-derived keratinocytes,16 and iPSC-derived keratinocyte and fibroblasts have the capacity to form well-differentiated skin constructs.15 With the possibility of producing iPSC-derived DP cells, and the development of new technologies and methods of transplantation, HFs engineered entirely from iPSC may become a possibility.
12.3 Methods of Delivery
12.3.1 Direct Injection
The simplest method of modifying the dermal milieu of balding scalp is by direct injection of cells or growth factors. For example, local injection of platelet-rich plasma (PRP), by itself or to supplement hair transplantation surgery, has been employed to address different forms of nonscarring alopecias, namely, AGA and alopecia areata. However, its efficacy needs to be confirmed in more high-quality randomized controlled studies.18
The promise of stem cell therapy has intrigued patients and physicians, and the as-yet unregulated practice of harvesting autologous stem cells, usually from the patient’s own adipose tissue, and injecting them into the scalp has grown in the last few years. However, it has been shown that these mesenchymal stem cells rarely persist in the recipient site, and their transient therapeutic benefit, if any, may be attributed to the paracrine action of growth factors and cytokines that they secrete. Indeed, cell-free conditioned media from these stem cells have been shown to have similar outcomes and may pave the way to understanding the many ways we can modify the dermal microenvironment to promote hair regeneration.19
Direct injection of dermal sheath cells has also been put forth as a possible treatment for AGA. Dermal sheath cells from the dermal sheath cup are microdissected from HFs obtained from a small punch biopsy and expanded in culture for several weeks before they are reintroduced into the reticular dermis. Since these cells are believed to be stem cells that replenish the DP,20 they are thought to rejuvenate the senescent DPs of miniaturized HFs to allow them to reenter the hair cycle. Phase I/II preliminary results of this treatment modality reported a modest increase in follicular density in treated sites of AGA patients, compared with control-treated sites.21
12.3.2 Bioengineered Human Hair Follicles
To date, several different strategies have been proposed to induce de novo hair formation in vivo using cultured cells. One recent study demonstrated, as a proof of concept, the generation of a 3D integumentary system containing HFs by using embryoid bodies of mouse iPSCs.22 Using this strategy with human iPSCs will provide an invaluable patient-specific source of fully-grown hair for hair transplantation surgery. However, the method used in this study requires transplantation of embryoid bodies into the kidney capsule of immunodeficient mice, which represents a considerable limitation for clinical translational studies, in addition to the aforementioned safety concerns of iPSCs. The use of animal hosts in this technique could potentially be eliminated in the future by using bioengineering techniques to recreate the physiological microenvironment in vitro.
Other investigators have developed a hair germ-like 3D culture method by mixing bulge region–derived epithelial cells and scalp HF–derived intact DPs.23 Intracutaneous transplantation of these cellular mixtures induced hair growth on mice. Despite the success of HF induction in this assay, as well as previous studies using intact human DPs, it has been challenging to achieve de novo hair formation using cultured human DPs as human DPs significantly lose their hair inductive characteristics after several passages in vitro. Our group recently demonstrated that 22% of intact DP gene signature can be restored through 3D spheroid culture of DPs, enabling hair induction in vivo from cultured human cells for the first time.6 While this finding taught us that the 3D conformation and microanatomy of the DP is essential in its function, it highlighted that simple spheroid culture could not restore the inductive efficiency of intact DPs. This limitation is primarily due to incomplete restoration of intact DP gene signature, which can be circumvented by leveraging master regulator transcription factors (identified using a systems biology approach) that govern the hair inductivity phenotype. Overexpression of these master regulator genes in combination with 3D spheroid culture may further restore the full hair inductive gene signature of DP cells, enhancing the efficiency of hair induction, thus making this technique more feasible for hair transplantation.
An alternative approach would be growing human hair in a fully ex vivo context. However, this has been a long-standing challenge for the field of hair regenerative medicine. Tissue-engineered human HFs can theoretically be achieved through directed differentiation of iPSCs into an integumentary organ or using a sufficient number of primary or iPSC-derived cell types to initiate cellular signaling necessary for HF neogenesis (e.g., DPs and KCs). Since these two approaches showed promising results in vivo as described earlier, the same could potentially be established in vitro by recreating the pivotal microenvironmental cues during folliculogenesis, and even hair cycling. One of the requirements in the iPSC-embryoid body approach is the expansion of the transplanted tissue, which is enabled by adequate vascularization at the transplantation site.22 Recently developed microfabrication techniques for tissue vascularization can allow for effective delivery of nutrients, leading to increased tissue sizes in vitro. Furthermore, other emerging engineering techniques, such as 3D printing and bioprinting, can be employed to control the spatial arrangement of cells in a biomimetic 3D matrix.17 Once established, tissue-engineered HFs can be delivered within engineered 3D skin constructs for skin replacement therapy, or directly transplanted as FUs onto patients, and will transform the future and potential of hair regenerative medicine.
12.4 Future of Cell Therapy
The future of therapy for AGA resides in the advancements that will be made in both autologous stem cell therapy and the development of drugs for hair growth stimulation. The improvement of personalized medicine technologies has shaped a novel way to approach hair transplant procedures, and stem cell cloning offers new possibilities beyond autologous transplants. In parallel, while this has significantly moved the field forward, the development of 3D skin models has become a prerequisite for better drug testing platforms. Achieving complete skin modeling in vitro will also advance the way hair loss or other skin diseases are treated (Video 12.1 and Video 12.2).
12.4.1 Personalized Medicine
Advances in cell culture techniques, iPSC technology, and tissue engineering methods have provided exciting new avenues for regenerative medicine. FUE allows easy small-scale removal of a FU without significant scarring. A combination of all these technologies means that patients could experience a streamlined process whereby a minimally invasive procedure to obtain autologous cells is followed by iPSC generation and reconstruction of HFs ex vivo, which are then transplanted in a specially designed (perhaps by algorithm) hairline to the patient’s own facial proportions. Attainment of these skills will also supplement the management of other conditions such as chronic wounds, second- and third-degree burns, and management of surgical wound closure.
12.4.2 Universally Compatible Stem Cell Lines
Currently, autologous stem cells are often used to treat patients with various diseases, and are generally FDA-approved because of their negligible immunogenicity and favorable safety profile. However, it is difficult and invasive to obtain adequate patient-specific autologous cells, and processing them into skin constructs or other autologous cell products is expensive and labor intensive. As such, patients usually have to wait for a long time before autologous products are available for treatment. Availability of allogeneic cell-based treatment can circumvent the limitations posed by autologous cells, because patients can have immediate access to off-the-shelf products and large-scale manufacture, and validation of allogeneic cell products reduces production costs and improves consistency and quality.
However, the human leukocyte antigen (HLA) mismatch between donor cells and the recipient’s immune cells can cause rejection of allogeneic tissue grafts.24 Immunosuppressive drugs can be used to reduce immune rejections by suppressing the body’s immune system, but these drugs are not always effective and can cause side effects.25
As discussed earlier, somatic cells from patients can be reprogrammed into stem cells by iPSC before being redifferentiated into various cell types for autologous therapeutic applications. Somatic cell nuclear transfer (SCNT)-based conversion of somatic cells to stem cells is another approach, which is achieved by transferring a human nucleus into a nucleus-free human egg.26 However, this methodology is technically challenging and costly. iPSC technology has clear advantages, but mutations and genetic instabilities associated with reprograming raise safety concerns.27,28,29 Establishment of a bank of hESCs has been proposed to provide patients with HLA-matched hESCs for regenerative applications.30 However, the polymorphism of HLA antigens, with more than 1 billion diploid combinations of HLA class I (HLA-I) genes alone,31 makes it unfeasible to establish such a ESC bank to include all the HLA polymorphisms for HLA matching. It is an appealing concept in the future to create a universally immune-compatible stem cell line that can be adopted for a wide range of clinical implications.32
12.4.3 Advances and Future of Skin Constructs
Using cells in 2D cultures also has limitations. The absence of the dermal microenvironment, stem cell niche, and mechanical cues can alter cell behavior and even gene expression. Therefore, 3D cultures have been the method of choice for recapitulating some of the in vivo context and bridging the gap between animal testing and clinical trials. A variety of 3D tissue models have emerged over the recent years, such as spheroids, organoids, hydrogels, organs-on-chip, and more recently 3D bioprinting. For skin, 3D human skin equivalents, made of a dermis-like structure (collagen matrix containing fibroblasts) topped by a stratified epidermis (keratinocytes), have significantly improved the possibility for ex vivo drug testing on human cells.
These 3D skin models can be developed to model AGA, as well as other diseases, and could be a powerful tool for drug screening. Currently, 3D disease modeling is limited to very few conditions,33 but the increasing number of studies to incorporate new knowledge and various cell types, along with the development of microfluidic platforms such as a skin-on-a-chip,34,35 will open new avenues for high-throughput, focused drug screening for dermatology.
Editor’s Comment
Dr. Christiano provides a fascinating view of the implementation of stem cells to halt hair loss or regrow hair for patients with various forms of alopecia. It is a fascinating field with many potential implications for the future. However, I would like to make a practical comment. Having been in the field for 28 years I can tell you that since the beginning I have been asked again and again “When are we going to be able to clone hair.” Often this question comes after another headline about a potential breakthrough seen in the news. I always have had to answer “Maybe in 5–10 years.” Twenty-eight years later I am still giving the same answer.
I am sure with the continued dedication of physicians such as Dr. Christiano that one day stem cell therapy will do miraculous things in the clinical world. However, as she has pointed out it is very complicated.
Insofar as the use of human stem cells for hair loss, we are learning that we still have a very long way to go before, using current technology, human stem cells can be coaxed into forming new terminal hairs for practical clinical application. This is true whether one is trying to clone hairs in vitro or create new hairs in vivo. This is also true whether the source of the stem cells is de-differentiated adult stem cells, stem cells from the stromal vascular fraction (SVF) of fat, or embryonic stem cells.
One thing that is apparent is that human stem cells when delivered to an area undergo degeneration and release chemical signals to other cells to come into the area. It is the other cells that are responsible for repair if it is to occur. When delivered to a site directly, the cells do not transform into the cell type of the surrounding cells.
When human stem cells have given rise to other cells, it has occurred in the context of a somewhat differentiated cell, for instance bone marrow–derived hematopoietic stem cells being used to repopulate bone marrow in someone who is a candidate for stem cell therapy for a lymphoma.
Recently, the focus of cell therapy for hair loss seems to have shifted from cloning new cells to using “multiple growth and signaling factors” within cells to stimulate the regeneration of preexisting miniaturized hair. PRP is an example of using this approach with the factors within platelets. Rather than using stem cells to clone or grow new hairs it may be that we should be searching for the factors within stem cells that can communicate to other cells the need for repair and recruitment of appropriate cells to perform these tasks.
Along these lines, several other areas of research appear to be possible modalities for stopping hair loss and regrowing hair.
A recent breakthrough has been the rediscovery of exosomes. Exosomes may prove enormously useful in treating many diseases and injuries. Exosomes are membrane-bound vesicles derived from endosomes within the cytoplasm of cells. They were discovered over 30 years ago. These membrane-bound vesicles were originally thought to carry waste products from the cell. More recently, it has become apparent that these vesicles carry many types of information that allow cell-to-cell communication. Not only are these vesicles secreted from cells but they also can interact with cells and deposit material into the communicating cell(s).
Exosomes from stem cells contain many “growth and signaling factors” that have been postulated to stimulate the regeneration of preexisting miniaturized hair. It has been postulated that by using the exosomes from stem cell cultures rather than the actual stem cells, you can deliver higher amounts of these factors that actually do the work with less risk. Some have called this acellular stem cell therapy.
There are other potential therapeutic ways these exosome could be used: exosomes could be placed into areas of hair loss and deliver medication, genes, gene editing complexes, and other cell factors that could favorably affect hair growth. Exciting developments have occurred with the use of CRISPR and mRNA inhibition. With CRISPR it may be conceivable to edit genes relevant to hair growth and hair loss. Using mRNA inhibition, it may be possible to control expression of genes involved in hair loss and hair growth.
The future of these novel methods to treat hair loss and regrow hair is just beginning. We are entering a new period of scientific advances and innovation in cellular biology.
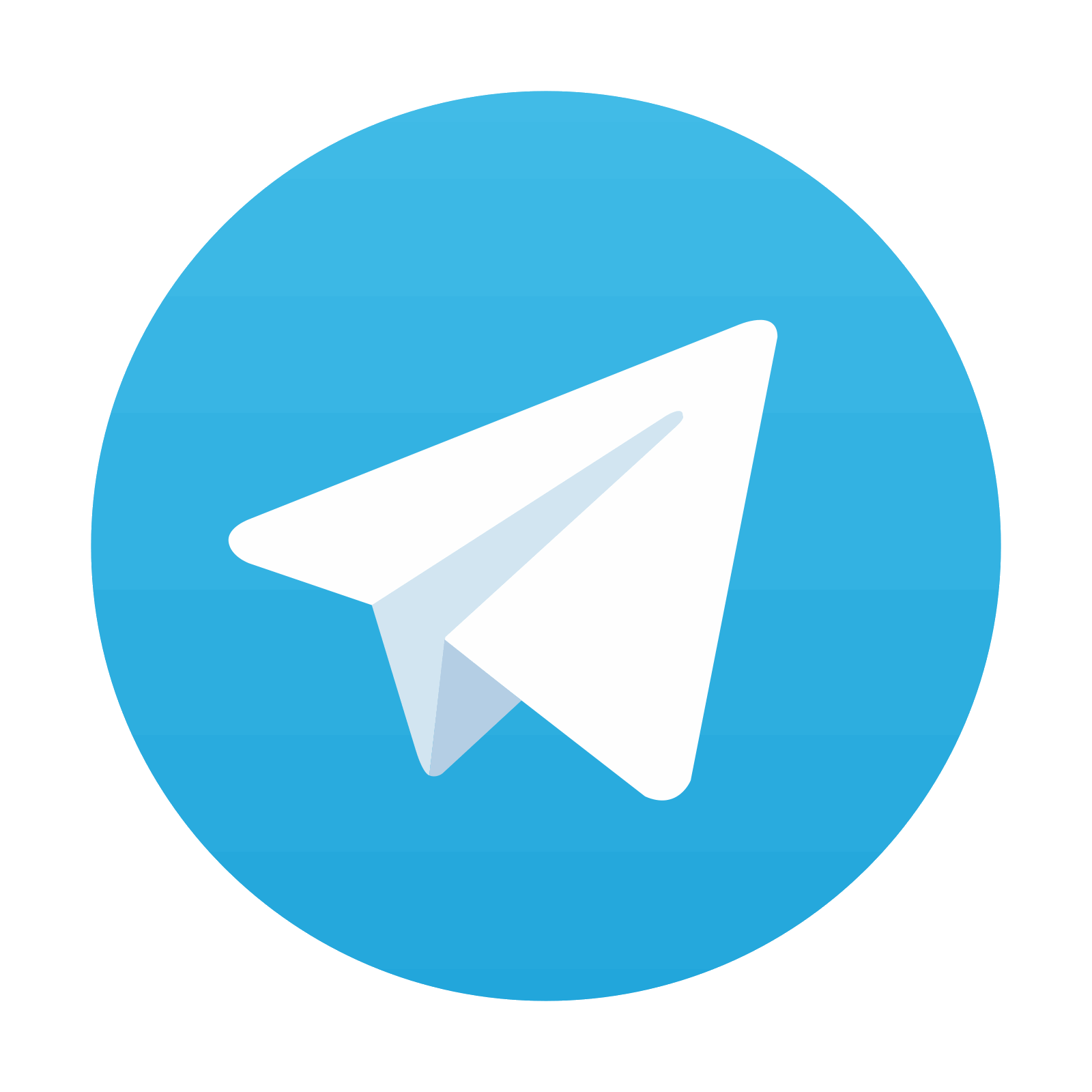
Stay updated, free articles. Join our Telegram channel
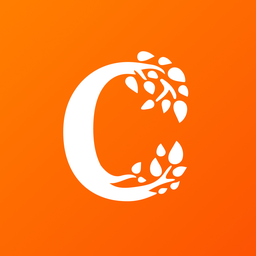
Full access? Get Clinical Tree
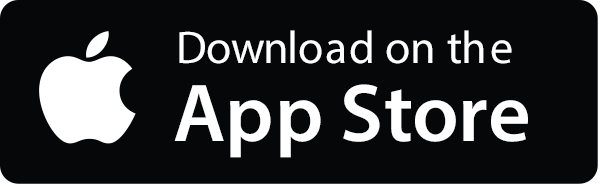
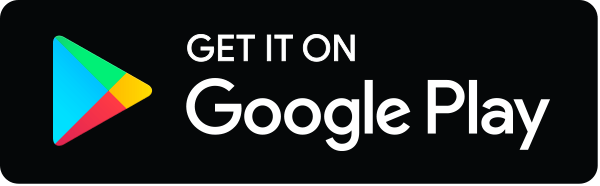