ACL Graft Healing and Biologics
Keywords
• Tendon-bone healing • Autograft • Allograft • Cytokine • ACL reconstruction
Key Points
Introduction
Operative reconstruction of a torn anterior cruciate ligament (ACL) has become the most broadly accepted treatment, aiming to restore native anatomy and a complete return to functional demands.1 Although the reported success rates of ACL reconstruction vary between 69% and 95%, return to previous activity level has recently been reported to be less than 50%.1–6 In addition, current surgical techniques have not been shown to prevent the development of late osteoarthritis after ACL injury.
An important, but underreported, outcome of ACL reconstruction is graft failure. Although this is a devastating outcome for the patient, treatment of failed primary ACL reconstructions also poses a challenge for the orthopedic surgeon. Basic science studies have shown that an anatomically placed graft sees forces that are similar to those of the native ACL and are substantially greater than the forces on a nonanatomically placed graft.7,8 The successful restoration of native knee kinematics through ACL reconstruction relies on the assumption that there is adequate healing of the graft/bone tunnel interface and reconstructed tissues to withstand the forces transmitted to the native ACL with athletic activity. With the well-established, inferior functional outcomes of revision ACL reconstruction compared with primary ACL reconstruction, prevention of graft failure should be the focus of early after-treatment.9,10 Recent efforts at advancing the clinical success of ACL reconstruction have focused on strategies to enhance and optimize the biologic environment of the graft-bone interface, promoting and potentially improving the healing rate and strength of the reconstruction.
The mechanism of graft failure is multifactorial in nature and may be attributed to either traumatic or nontraumatic causes. Recurrent trauma, resulting from early aggressive rehabilitation, a premature return to play, or reinjury of a well-healed graft can lead to graft failure. In addition, nontraumatic causes may include technical error in tunnel placement, occult limb malalignment or ligamentous laxity, failure of fixation, or poor biological incorporation.10 Often, multiple factors may be present to variable degrees. Biomechanical testing has shown that the time-zero strength of the most common graft choices is greater than the native ACL.11,12 Rather, the weakest link after ACL reconstruction is not the graft but the fixation points on the tibial and femoral side until the graft has adequately healed in the bone tunnel.13–16 The gradual healing at the enthesis and the intra-articular ligamentization process together make up the 2 main sites of biological incorporation after ACL reconstruction.17 An understanding of the tendon-bone healing and the intra-articular ligamentization process is crucial for orthopedic surgeons to make appropriate graft choices and to be able to initiate optimal rehabilitation protocols after surgical ACL reconstruction.
Graft-tunnel healing is influenced by many different factors, including surgical technical variables such as graft placement, graft length within the bone tunnel, graft fixation, graft tensioning, and graft-tunnel micromotion. These variables may even vary for different regions of the bone tunnels.18,19 However, the principal form of healing that occurs in the bone tunnels is primarily dependent on the graft used. Grafts with a bone plug (eg, bone-patellar tendon-bone graft, bone-quadriceps tendon graft) rely on bone-to-bone healing, whereas soft tissue grafts rely on tendon-bone healing, a slow process that does not recapitulate the native anatomy of the ACL insertion with regard to morphology or mechanical strength.20,21 However, all grafts depend on tendon-to-bone healing at the intra-articular tunnel apertures.22 Tissue engineering and biomechanical stimulation approaches to enhance this healing process have shown promising results in animal studies and include, but are not limited to, the use of a fibrin clot, platelet rich plasma, growth factors, stem cells, scaffolds, periosteum graft augmentation, bisphosphonates, autologous ruptured tissue, and mechanical loading.
Tendon-bone healing: autograft
Healing of a tendon graft inside a bone tunnel is particularly challenged by the complex transition site, which must allow for load transfer between the 2 distinct, inhomogeneous tissues: tendon and bone.23 Moreover, this transition site is markedly different from the load-transferring architecture of the insertion site of the native ACL.
The native ACL inserts into the bone through an insertion site that changes from ligament to bone directly and functions to transmit complex mechanical loads. This insertion site is made up of highly specialized tissue that gradually changes from ligament to bone through 4 zones: ligament, unmineralized fibrocartilage, mineralized fibrocartilage, and bone (Fig. 1).24 The increase of tissue stiffness along the insertion site from tendon to bone is likely controlled by collagen fiber alignment and a gradual increase of mineralization.25 A direct insertion from ligament to bone as the ACL has different from other ligaments and tendons, such as the medial collateral ligament (MCL), that run parallel to the bone and insert through an indirect insertion site. Indirect insertion sites do not gradually change from ligament to bone, but rather consist of specialized collagen fibers, called Sharpey fibers, which are oriented obliquely to the long axis of the bone and ligament and provide anchorage between the 2 tissues.
With anatomic ACL reconstruction, the native insertion sites are restored with respect to the insertion site locations on the femur and tibia.26,27 However, because the grafts are placed in bone tunnels, the structure and composition of the direct insertion site are not reproduced. Instead, the graft heals with a fibrovascular scar at the graft-tunnel interface and forms perpendicular collagen bundles to counteract the shear stresses, attaching the tendon to the bone.13,15,28 These perpendicular bundles resemble the Sharpey fibers of an indirect insertion site.13,15 The formation of these fibers begins at 3 to 4 weeks after graft placement and their size and number are positively correlated with the graft pull-out strength.13,15,28 Sharpey-like fibers continue to be present at 1 year after surgery while gradual osseointegration occurs, improving the graft attachment and incorporating the graft into the surrounding bone.13
The earliest phase of healing comprises an inflammatory response characterized by the presence of distinct subpopulations of macrophages. Around 4 days after surgery, macrophages recruited from the circulation and neutrophils are present in the healing tendon-bone interface and are involved in phagocytizing cellular debris, recruiting additional inflammatory cells, and assisting in proinflammatory cytokine release. After 10 days, resident, progenerative macrophages can be identified.29 These inflammatory cells repopulate the graft and produce numerous cytokines, like transforming growth factor β (TGF-β), which contribute to the formation of the fibrovascular scar tissue interface between the graft and the bone tunnel (Fig. 2).29 However, the formation of this scar tissue results in a mechanically inferior tendon-bone interface, with less organized collagen deposition and decreased pull-out strength than when this scar formation is diminished by macrophage depletion in animal models (Fig. 3).30 Macrophages, TGF-β, and the cyclooxygenase 2 (COX-2) pathway have a complex interplay, and although a depletion of macrophages may be accomplished by inhibiting the COX-2 pathway,31 different studies have shown that selectively inhibiting the COX-2 pathway delays healing with significantly decreased load to failure.32,33
During the first 8 weeks postoperatively, the tendon-bone interface undergoes significant immunohistologic changes. There is an infiltration and recruitment of macrophages and marrow-derived stem cells to the interface. Initially, the interface consists of mainly granulation tissue containing type III collagen and produces growth factors like vascular endothelial growth factor (VEGF) and fibroblast growth factor (FGF), stimulating angiogenesis and enlarged fibroblasts at the healing enthesis. Simultaneously, like fractured bone, the bone tunnel starts a process of endochondral ossification and chondroid cells appear from the tunnel wall, which degrade the granulation tissue and produce type II collagen. Gradually, the granulation tissue is replaced by maturing lamellar bone and the chondroid cells decrease in number. Meanwhile, shear stresses between the tunnel and the graft cause Sharpey-like fibers to develop, and from the margins of the hypocellular graft, FGF is expressed, attracting fibroblasts to incorporate into the graft and produce type III collagen.33
Although the inflammatory phase is essential for the tendon-to-bone healing process, the phase should be transient and gradually progress to the proliferation phase. Early return to sports or an aggressive rehabilitation protocol may lead to micromotion of the graft inside the tunnel.34 This relative movement between graft and tunnel may impair healing because of continuous microtrauma of the healing tissues, which results in a sustained inflammatory response at the healing enthesis site. The graft-tunnel motion may also lead to osteoclast activation, which stimulates tunnel widening by bone resorption.34,35 Although excessive motion impairs healing, some controlled loading has shown to be beneficial in tendon healing (Fig. 4).36 Controlled mechanical loads after a delay to allow resolution of acute postoperative inflammation result in improved mechanical and biological parameters.37,38
During the proliferation phase, the stem cells proliferate and differentiate, and matrix metalloproteinases (MMPs) and serine proteases degrade the provisional matrix. The healing cells synthesize and deposit new extracellular matrix with progressive bone ingrowth, which results in an improved load-to-graft failure.13,39 Gradually, remodeling of the newly formed matrices takes place; the newly formed woven bone, interface tissue, and graft remodel, with establishment of collagen fiber continuity between tendon graft and bone, and cellularity and vascularity at the interface decrease (Fig. 5).
Tendon-bone healing: allograft
The use of allograft for soft tissue reconstructive surgery has increased significantly in recent years. The main advantages of using an allograft for ACL reconstruction include the ease of customizing the graft to the desired size and shape, availability, lack of donor-site morbidity, reduced operation times, reduced postoperative pain, and a theoretically lower risk of arthrofibrosis.40,41 Moreover, some studies have reported no significant difference in clinical outcomes for bone-patellar tendon-bone autografts and allografts, although others have reported significantly greater failure rates in younger patients less than the age of 40 years.42–44
Allografts heal through the same biologic pathway of creeping substitutionlike autografts, but at a slower rate. The intra-articular portion of the allograft heals by acting as a type I collagen scaffold, which is populated from the tunnels with host cells derived from the bone and synovial fluid.45 Angiogenesis is predominately facilitated from the infrapatellar fat pad distally and from the posterior synovial tissues proximally.46 This angiogenesis is followed by invasion of fibroblasts and synovial cells to repopulate the tendon with host cells and gradually incorporate and remodel the matrix of the graft. As remodeling of the matrix takes place, the tensile strength of the graft is substantially reduced initially and then increases as remodeling is finalized. Allografts lose more of their initial strength during remodeling than autografts, rendering the grafts particularly vulnerable to failure in the subacute phase of postoperative rehabilitation and with a premature return to sport.47
Even although ligaments and tendons are relatively hypocellular, the use of fresh grafts might stimulate the host immune response by lymphocyte invasion, perivascular cuffing, and eventually graft rejection.48 This immune response is elicited mostly by the expression of major histocompatibility antigens, present on the surface of viable allograft cells. Therefore, fresh tendon allografts are generally not used. Although sterilization can decrease the presence of viable allograft cells, this treatment comes at the expense of decreased biomechanical strength of the graft, which is associated with irradiation or chemical processing.
Tendon allografts can be sterilized by several methods, including chemical sterilization, deep freezing, or γ-irradiation, resulting in decreased graft immunogenicity and reduced risk of disease transmission. However, each method has its own drawbacks, which should be taken into consideration when performing ACL reconstruction with allograft. Chemical sterilization may result in inflammatory reaction, delayed remodeling, and inferior mechanical strength and may not be ideal for soft tissue allograft.49 Deep freezing is an effective method to kill the donor cell, but may still result in a detectable immune response directed to the matrix antigens, which can also influence graft incorporation and remodeling.50
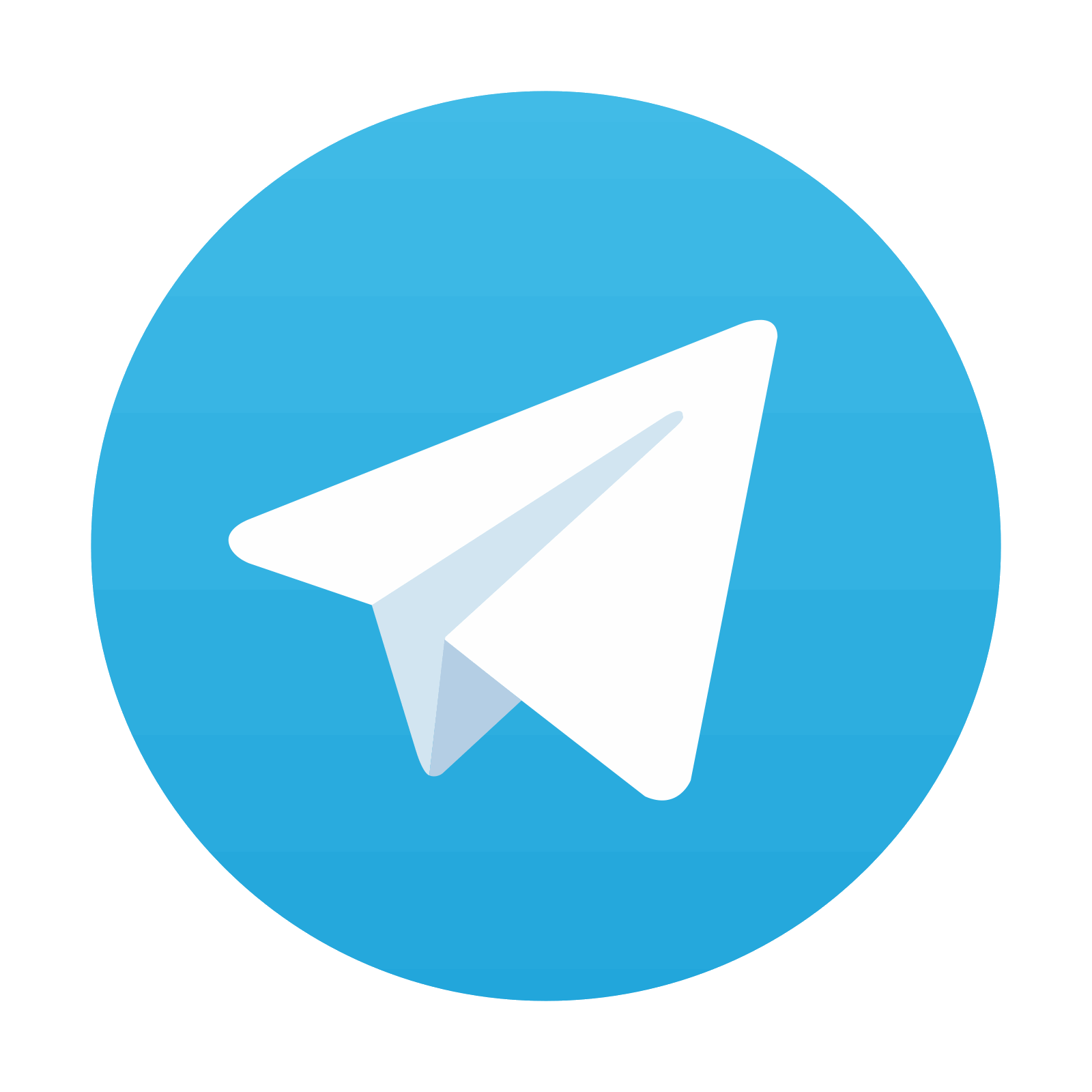
Stay updated, free articles. Join our Telegram channel
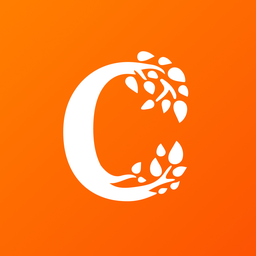
Full access? Get Clinical Tree
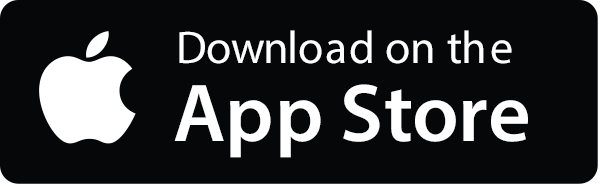
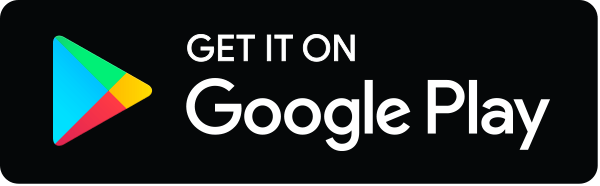