Phototherapy is not new! It was being used more than 4,000 years ago
Light-emitting diodes have attracted interest as a phototherapeutic source
LEDs are solid state and robust
LEDs are comparatively inexpensive
History of Phototherapy
Phototherapy in its broadest sense means any kind of treatment (from the Greek therapeia ‘curing, healing,’ from therapeuein ‘to cure, treat.’) with any kind of light (from the Greek phos, photos ‘light’). The modern accepted definition of phototherapy, however, has become accepted as: “the use of low incident levels of light energy to achieve an athermal and atraumatic, but clinically useful, effect in tissue”. Under its basic original definition, phototherapy is an ancient art because the oldest light source in the world is the sun, and therapy with sunlight, or heliotherapy, has been in use for over 4,000 years with the earliest recorded use being by the Ancient Egyptians.1 They would treat what was probably vitiligo by rubbing the affected area with a crushed herb similar to parsley, then expose the treated area to sunlight. The photosensitizing properties of the parsley caused an intense photoreaction in the skin leading to a very nasty sunburn, which in turn hopefully led to the appearance of postinflammatory secondary hyperpigmentation, or ‘suntan’ thereby repigmenting the depigmented area. In their turn the Ancient Greeks and Romans used the healing power of the sun, and it was still being actively used in Europe in the eighteenth, nineteenth and early twentieth century, particularly red light therapy carried out with the patient placed in a room with red-tinted windows. One famous patient was King George III of Great Britain and Northern Ireland who ruled from 1760 to 1801, popularly though erroneously known as ‘Mad King George’. We now strongly suspect that he was actually suffering from the blood disease porphyria, so being shut in a room with red-draped walls and red tinted windows to treat his depression probably only served to make him even more mad, since porphyria is often associated with severe photosensitivity! Entities treated this way included the eruptive skin lesions of rubella and rubeola, and even ‘melancholia’, as was the case with King George III, now recognised as clinical depression. Hippocrates, the Father of Medicine, certainly concurred with the latter application some two millennia before King George: Hippocrates prescribed sunlight for depressive patients and believed that the Greeks were more naturally cheerier than their northern neighbors because of the greater exposure to the sun.
In the field of wavelength-specific phototherapy research, red light therapy was examined at a cellular level under the newly-invented microscope by Fubini and colleagues in the late eighteenth century,2 who were able to show that visible red light, provided via lenses and filters from sunlight, selectively activated the respiratory component of cellular mitochondria. There is nothing new under the sun. However, the sun is a fickle medical tool, particularly in northern Europe, and modern phototherapy as we know it started around the turn of the last century with Finsen’s electric arc lamp-based system, giving phototherapy at the turn of a switch, independent of the sun.3 However, apart from the use of blue light therapy for neonatal bilirubinemia which continues to the present day, phototherapy was, in the majority of its applications, overtaken in the first part of the twentieth century by better medication or improved treatment techniques.
The development of the first laser systems, a race which was narrowly won by Theodore Maiman in 1960 with his flashlamp-pumped ruby-based laser, next gave clinicians and researchers a completely different and unique light source to play with. In the 4 years between 1960 and 1964, the ruby laser was followed by the argon, helium-neon (HeNe), neodymium: yttrium-aluminum-garnet (Nd:YAG) and carbon dioxide (CO2) lasers all of which have remained as workhorses in the medical field, and the HeNe laser (632.8 nm) has in fact provided a large bulk of the phototherapy literature over the last three decades. As for light-emitting diodes (LEDs), the first light from a semiconductor was produced in 1907 by the British experimenter H. J. Round. Independently in the mid 1920s, noncoherent infrared light was produced from a semiconductor (diode) by O-V Losev in Russia. These studies were published in Russia, Germany and the UK, but their work was completely ignored in the USA.4 It was not till 1962 that the first practical and commercially-available visible-spectrum (633 nm, red) LED was developed in the USA by Holonyak, regarded as the ‘Father of the LED’ while working with the General Electric Company. In the next few years, LEDs delivering other visible wavelengths were produced, with powers ten times or more that of Holonyak’s original LED. For reasons which will be discussed later, these LEDs were really inappropriate as therapeutic sources, although they were extremely bright and very cheap compared with laser diodes, and it was not till the late 1990s that a new generation of extremely powerful, quasimonochromatic LEDs was developed by Whelan and colleagues as a spin-off from the National Aeronautic and Space Administration (NASA) Space Medicine Program.5 Unlike their cheap and cheerful predecessors, the so-called ‘NASA LEDs’ finally offered clinicians and researchers a new and truly practical therapeutic tool.6
The What and Why of LEDs
What Is an LED?
Light-emitting diodes belong to the solid state device family known as semiconductors. These are devices which fall somewhere between an electrical conductor and an insulator, although when no electrical current is applied to a semiconductor, it has almost the same properties as an insulator. Simply explained, light-emitting semiconductors or diodes consist of negative (N-type) and positive (P-type) materials, which are ‘doped’ with specific impurities to produce the desired wavelength. The n-area contains electrons in their ground or resting state, and the p-area contains positively charged ‘holes’, both of which remain more or less stationary (Fig. 1a–c). When a direct current electric potential with the correct polarity is applied to an LED, the electrons in the N-area are boosted to a higher energy state, and they and the holes in the P-area start to move towards each other (Fig. 1d), meeting at the N/P junction where the negatively-charged electrons are attracted into the positively-charged holes. The electrons then return to their resting energy state and, in doing so, emit their stored energy in the form of a photon, a particle of light energy (Fig. 1e). The wavelength emitted is noncoherent, ideally very narrow-band, and depends on both the materials from which the LED is constructed, the substrates, and the p-n junction gap. Table 1 shows a list of the main substrates and associated colors. Figure 2 shows the anatomy of a typical dome-type LED. These can be mounted on circuit boards at regular and precise distances from each other to provide an LED array, part of which is shown in Fig. 3. However, the latest generation of LEDs actually form part of the board (so-called ‘on-board’ chips) which are much more compact than the dome-type LED and more efficient.
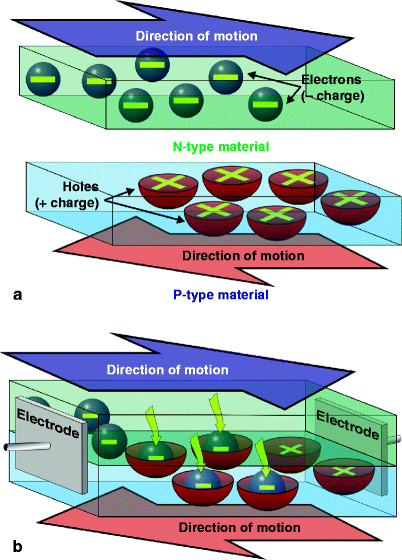
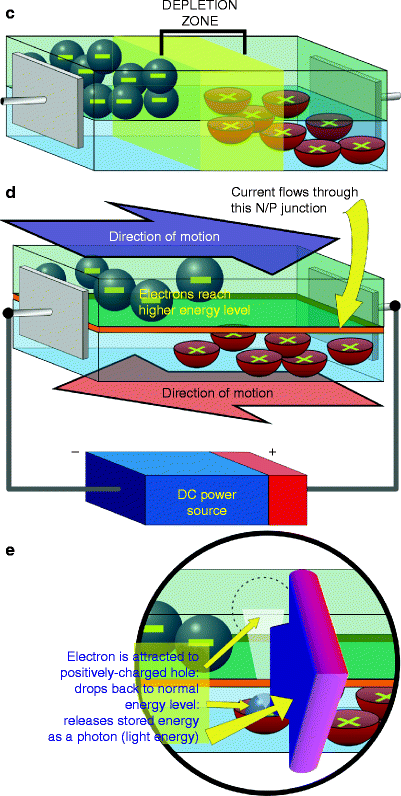
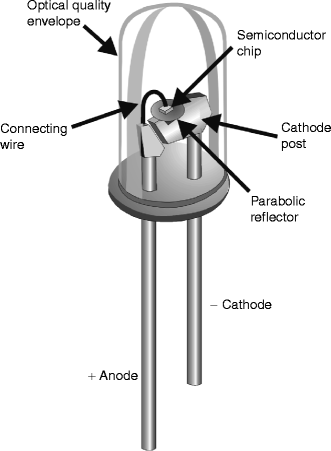
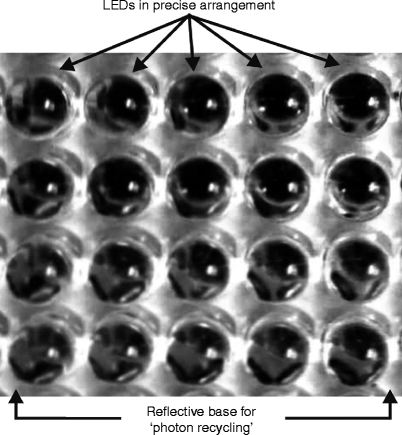
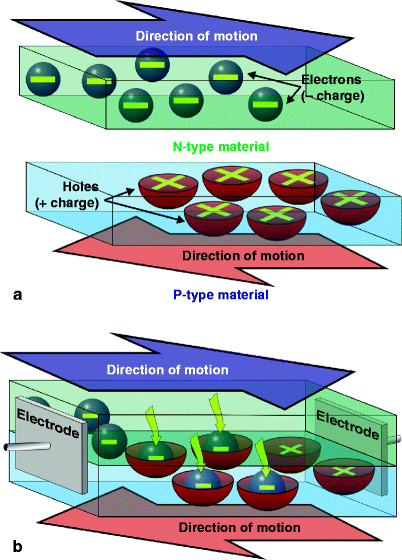
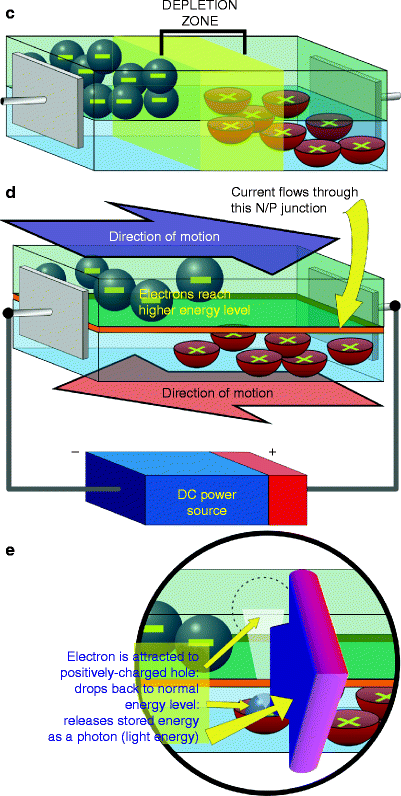
Fig. 1
What is an LED and how can it produce light? (a) An LED is basically composed of two materials, the N-type or negative material and the P-type or positive material. The N-material contains negatively charged electrons which move as shown, and the P-material contains positively charged holes, which move in the opposite direction. When the materials are apart and not connected to any power source, movement continues, so both materials are conductors. (b) When the materials are sandwiched together, however, without any power applied to the electrodes attached to opposite ends, the negatively charged electrons in the center of the chip are attracted to the holes, and form an area called the depletion layer as seen in (c) and all movement ceases in both the N- and P-materials: the chip is now an insulator. (d) Power is applied to the electrodes, with the positive electrode or anode at the origin of movement of the holes and the negative electrode or cathode at the origin of movement of the electrons. Observing the polarity when connecting a direct current (DC) power source is extremely important. Power flows through the junction between the materials, called the N/P junction, and movement of both electrons and holes starts again, but with power applied the electrons move to a higher energy level from their ground or resting state. (e) As in 1b above, the N-electrons are attracted to the P-holes, but in moving down through the N/P junction they must return to their ground energy level, and lose their extra stored energy in the form of a photon, the smallest packet of light energy. Unlike the situation in 1b, however, when power is applied this action continues endlessly and no depletion layer is formed. The N- and P-materials are ‘doped’ with other materials which determine the distance of the ‘fall’ between electrons and holes: the greater the distance the electrons have to fall, the higher is the energy level of the photons emitted. Photons with high energy levels have shorter wavelengths than those with lower energy levels, thus the wavelengths of the emitted light are determined by the materials and their doping. High quality N- and P-materials and pure doping substances will give photons of very nearly the same wavelength, i.e., quasimonochromatic light
Table 1
Most common substrate combinations and the colors they are capable of producing
Substrates | Formula | Colors produced |
---|---|---|
Aluminum gallium arsenide | (AlGaAs) | Red, infrared |
Aluminum gallium phosphide | (AlGaP) | Green |
Aluminum gallium indium phosphide | (AlGaInP) | Green, yellow, orange, orange-red(all high-intensity) |
Gallium arsenide phosphide | (GaAsP) | Yellow, orange, orange-red, red |
Gallium phosphide | (GaP) | Green, yellow, red |
Gallium nitride | (GaN) | Blue, green, pure green (emerald green): also white (if it has an AlGaN Quantum Barrier, so-called ‘white light’ LED) |
Indium gallium nitride | (InGaN) | Near ultraviolet, blue, bluish-green |
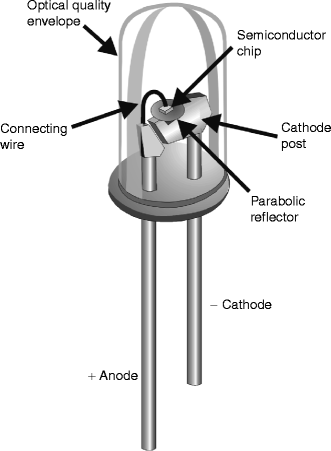
Fig. 2
Anatomy of a typical high-quality dome-type LED. The cathode is always shorter than the anode and there is a flat surface in the base of the LED by the cathode so polarity is clearly determined when connecting to a DC power source. On top of the cathode post and forming part of the negative electrode of the LED chip is a parabolic reflector in which the chip itself is mounted thus ensuring as much light as possible is directed forwards, with a consistent angle of divergence, typically 60° steradian or less depending on the specifications of the LED. A fine wire connects the positive electrode of the chip to the anode post, thus completing the circuit. The entire assembly is encapsulated in an optical quality clear plastic envelope, giving the final assembly its robust nature
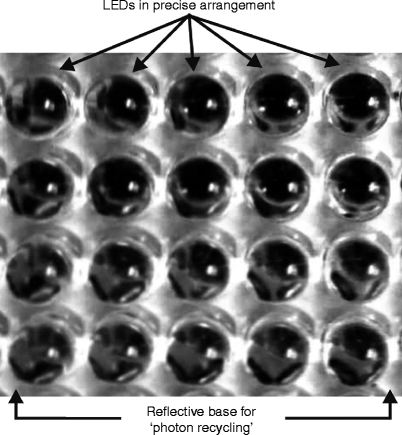
Fig. 3
Close-up view of dome-type LEDs mounted in an actual array from a therapeutic system. Note the precise x-y spacing of the LEDs, (cf Fig. 6 and associated text), and the reflective backing into which they are mounted. When light is incident on living skin, a certain amount will be reflected from the outer layer of skin, the stratum corneum. The longer the wavelength, the greater this reflection will be. In addition, some light is always back-scattered out of skin. The purpose of the reflective backing of the array is to capture these photons and reflect them back into the skin, known as ‘photon recycling’
What Is the Difference Between LEDs and Lasers or IPLs?
The laser is a unique form of light energy, possessing the three qualities of monochromaticity, collimation and phase which make up the overall property of ‘coherence’. Monochromaticity means all the photons are of exactly the same wavelength or color; collimation means the built-in parallel quality of the beam superimposed by the conditions of the laser resonator; and phase means that all of the photons march along together exactly equidistant from each other in time and in space. Laser diodes do not have inherent collimation, but because they are still true lasers, and therefore a so-called point source, the light can be gathered and optically collimated: the humble but ubiquitous laser pointer works on this principle. Intense pulsed light is, on the other hand, totally noncoherent, with a very large range of polychromatic (multicolored) light from near infrared all the way down to blue; has no possibility of collimation with extreme divergence; and has its vast variety of photons totally out of phase. The new generation of LEDs, on the other hand, has an output plus or minus a few nanometers of the rated wavelength, and so are classed as quasimonochromatic; some form of optical collimation can be imposed on the photons which are divergent but do have some directionality; but they are not in phase. Laser energy can easily produce high photon intensity per unit area, IPLs much less so, but provided LEDs are correctly arrayed, they are capable of almost laser-like incident intensities. Figure 4 schematically illustrates the differences between lasers, IPLs and LEDs. In short, LEDs for therapeutic applications must be quasimonochromatic, be capable of targeting wavelength-specific cells or materials, have stable output, and be able to deliver clinically useful photon intensities.
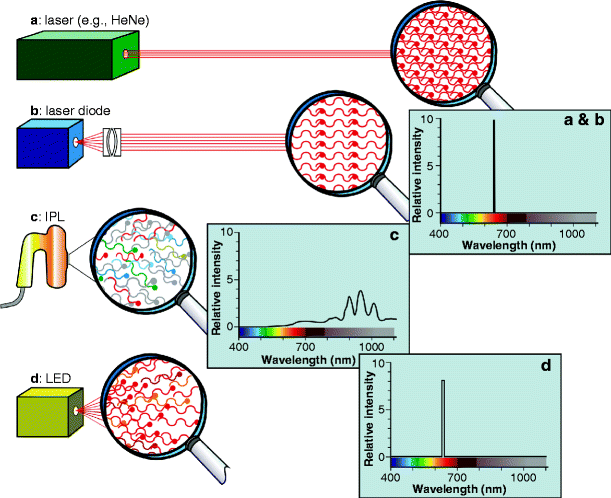
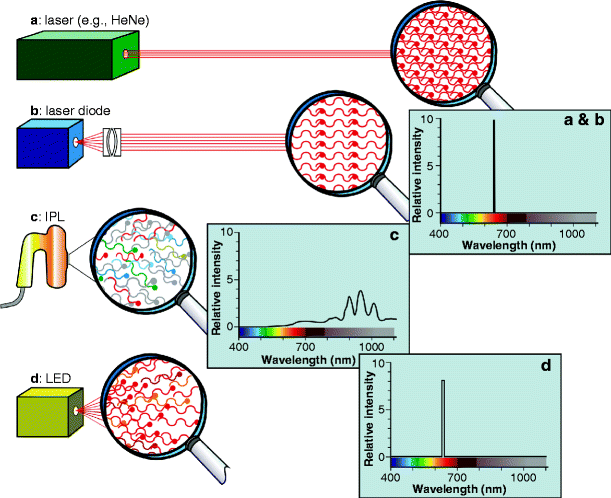
Fig. 4
Comparison among the output characteristics of a laser, laser diode, intense pulsed light system and a new generation LED. (a) A laser emits all of its energy at one precise wavelength, in a coherent beam, i.e., monochromatic, collimated and with the photons all in phase both temporally and spatially. If a ‘special magnifying glass’ could view the beam, it would show the picture as seen in the figure. All of the energy is delivered at a precise wavelength, as illustrated in the spectrogram, so the relative intensity of the beam is extremely high. (b) A laser diode has all the characteristics of a laser, except that the beam is divergent, without collimation. However, because it is a point source the beam can be collimated with condensing optics. The magnified view of the beam shows a lower photon intensity than the laser, but the relative intensity is still very high. (c) An IPL system emits a pulse of broad-band polychromatic noncoherent light, so the ‘magnifying glass’ would show a plethora of widely divergent photons of many different wavelengths, but with the majority in the near infrared as seen from the spectrogram. Because of the very broad waveband, the relative intensity at any given wavelength is low to very low. (d) The LED is somewhat similar to the laser diode, but the light is noncoherent, highly divergent and quasimonochromatic. The ‘magnifying glass’ shows plenty of photons, mostly the same color, with some degree of directionality but without the true collimation and phase associated with the laser diode. The relative intensity is still very high, however, because the vast majority of the photons are being delivered at the nominal wavelength with a very narrow waveband of plus or minus a very few nanometers
Why Use LEDs?
There are many excellent laser and intense pulsed light (IPL) systems available to the dermatologist. Why should LEDs be considered as a viable alternative phototherapy source? The main reasons are efficiency and price. The electricity-light conversion ratio of a typical laser is very low, requiring 100 or even 1,000 of watts in to give an output of a few watts. The same applies to IPL systems, where the flashlamp has to be pumped with enormous amounts of energy to provide polychromatic light, which may however be filtered (cut-on or cut-off). Even when filtered, IPL energy is delivered over a waveband rather than at a specific wavelength. In the case of LEDs, which are quasimonochromatic and require no filtering, the conversion efficiency is very high so that very few watts at a low voltage are required to produce a clinically useful output. LEDs are much less expensive than even laser diodes. Depending on quality and wavelength, anywhere from 300 new-generation LEDs can be purchased for the cost of a single laser diode. The cost of laser and IPL systems is very high, so a much cheaper LED-based system offers the possibility to halt the ever-upward spiralling costs of health care for both the clinicians and their patients. A further advantage is the solid state nature of LEDs. There are no filaments to be heated up, and no flashlamps are required to produce light or to pump the laser medium: LEDs thus run much cooler than their extremely higher-powered cousins, so less is required in the way of dedicated cooling systems, again helping to reduce the cost. However, some cooling of LEDs is still required, especially when LEDs are mounted in multiple arrays, because as the temperature of an LED increases, its output will move away from the rated wavelength. When wavelength cell- or target-specificity is required, this could be a major problem. The solid state nature of LEDs also makes them much more robust than either lasers or IPL systems, so they tend to be able to take the sometimes not-so-gentle handling which is part of a busy clinical practice without causing either output power loss or alignment problems. Finally, LEDs can be mounted in flat panel arrays, which may in turn be joined together in a treatment head which can be adjustable to fit the contour of the large area of tissue being treated, whether it is the face, an arm, the chest or back, or a leg. Compare this potentially very large treatment area of some 100 of square centimeters with that of a laser, usually a very few millimeters in diameter, or that of an IPL treatment head, typically 1 cm × 3 cm, and the clinician-intensive nature of the latter two is quickly evident when large areas are to be treated such as the entire face. Multiple shots are required, and the handpiece has to be manually applied and controlled by the user. The LED-based treatment head can be attached to an articulated arm to make individual adjustment even easier. Heads with different wavelengths can be designed to be easily interchangeable, controlled by the same base unit. With ‘set-it-and-forget-it’ microprocessor-controlled technology, the clinician simply sets the head up over the area to be treated, following the manufacturer’s recommendations, turns the system on, and he or she can then leave the patient for the requisite treatment time and attend to other patients or tasks. Moreover, in many cases a suitably trained nurse can carry out the treatment once the clinician has prescribed it, because LED systems are much more inherently safe for the patient than lasers or IPLs.
Basics of Light-Tissue Interaction
Light-emitting diodes provide athermal and atraumatic photoactivation LEDs are a viable and valuable phototherapeutic tool LEDs are capable of interesting light-tissue interactions, provided certain criteria are met. The most important criteria are: Wavelength. Determines both the target and the depth at which the target can be reached Quasimonochromaticity is essential Wavelengths should be applied separately and sequentially, and not combined at the same time Photon density. Gives suitably high intensity at all levels of target cells or materials Ensures sufficient athermal energy transfer to raise targets’ action potentials Dosimetry. Provided the wavelength and intensity are appropriate, correct dosage obtains the optimum effect with the shortest irradiation time Temporal beam profile. Continuous wave would appear to be more efficient for most cell types in vivo, compared with ‘pulsed’ (frequency modulated) light |
The main purpose of using phototherapy is to achieve some kind of clinical reaction in the target tissue through the use of light energy. If the incident power is high, heat will be the end product as with the surgical laser. If a too-low photon intensity is delivered, there will be very little or no reaction. The trick in LED phototherapy is to deliver just the right amount of photon intensity to achieve the desired clinical effect but in an athermal and atraumatic manner.
Photothermal and Athermal Reactions
Despite their very different output powers, lasers, IPLs and LEDs all depend on the ‘L’ which is found in all their names, standing for ‘light’. It could be said that they are all different facets of the same coin, but even in photosurgery, phototherapy plays a very important role. If we consider the typical beam pattern of a surgical CO2 laser in tissue, we see the range of temperature-dependent bioeffects as illustrated schematically in Fig. 5, ranging from carbonization above 200°C, vaporization above 100°C, through coagulation around 60–85°C, all the way down to photobiomodulation, which occurs atraumatically when there is no appreciable rise in the tissue temperature at the very perimeter of the treated area. These effects occur virtually simultaneously as the light energy propagates into the target tissue with photon intensity decreasing with depth, and can be divided as shown into varying degrees of photosurgical destruction and reversible photodamage, and athermal, atraumatic photobiomodulation. The zones are also shown in a typical CO2 laser specimen stained with hematoxylin and eosin (Fig. 5).
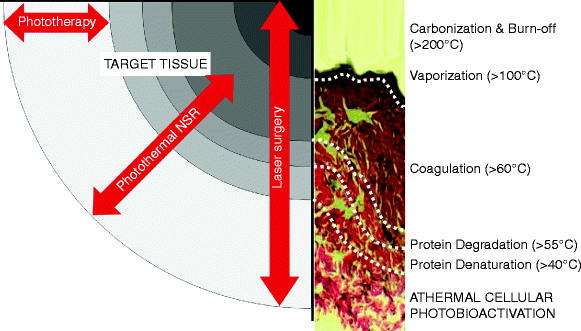
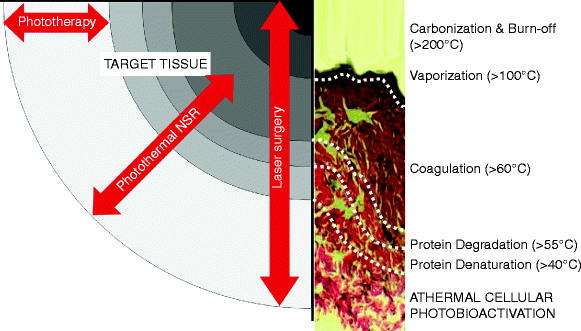
Fig. 5
Range of photothermal and athermal photobioreactions in tissue following a typical surgical laser impact, e.g., a CO2 laser. A hematoxylin and eosin stained specimen of actual CO2 laser treated skin is also included to show the typical histopathological changes for each of the bioreactions: the epidermis has been totally vaporized leaving a layer of carbon char above the coagulated dermis. The outermost layer, the photobioactivation layer, shows normal tissue architecture, even though some photons will have reached this layer and transferred their energy to the cells in an athermal and atraumatic manner. Laser surgery involves all levels of bioreactions. Photothermal nonablative skin rejuvenation (NSR) delivers controlled coagulative photothermal damage, with all the subsequent layers, whereas phototherapy only delivers athermal and atraumatic photobioactivation
Laser surgery involves all zones, but the importance of the photobiomodulative zone cannot be stressed enough. It is the existence of this zone which sets laser surgery apart from any other thermally-dependent treatment, such as electrosurgery, or even athermal incision with the conventional scalpel, and it is the photoactivated cells in this zone which provided the results that interested the early adopters of the surgical laser compared with the cold scalpel or electrosurgery, namely better healing with less inflammation and much less postoperative pain. IPL systems, and so-called nonablative lasers, produce areas of deliberate but controlled coagulative damage beneath a cooled and intact epidermis (Fig. 6), however they also produce the photobiomodulative zone to help achieve the desired effect of neocollagenesis and neoelastinogenesis through the wound healing process in the dermal extracellular matrix (ECM). LED-based phototherapy systems, on the other hand, deliver only athermal and atraumatic effects, but are still capable of inducing the wound healing process almost as efficiently as IPLs and nonablative lasers, as will be shown in detail in a later section.
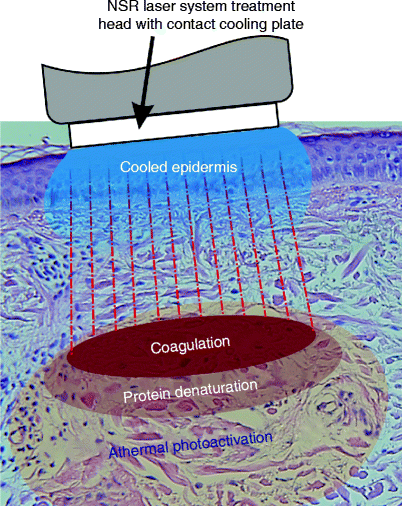
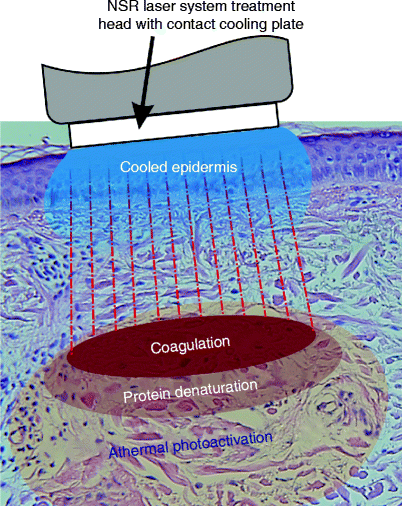
Fig. 6
Theory behind photothermal nonablative skin rejuvenation: the laser energy passes through the cooled epidermis without harming it, and delivers a controlled area of coagulation in the typically elastotic dermis associated with photoaged skin. However, the photons do not stop there, and there are zones of protein denaturation and, most importantly for the good result, athermal and atraumatic photoactivation around and beyond the controlled thermal damage. The photoactivated cells in the last of these three zones will assist with the wound healing process
Wavelength and Its Importance
The first law of photobiology, the Grotthus-Draper Law, states that only energy which is absorbed in a target can produce a photochemical or photophysical reaction. However, any such reaction is not an automatic consequence of energy absorption. It may be simply converted into heat, as in the surgical and non-ablative lasers or IPL systems, or re-emitted at a different wavelength (fluorescence). The prime arbitrator of this ‘no absorption-no reaction’ precept is not the output power of the incident photons, but their wavelength, and this comprises two important considerations: wavelength specificity of the target, or the target chromophore; and the depth of the target. Based on these two considerations, the wavelength must not only be appropriate for the chosen chromophore, but it must also penetrate deeply enough to reach enough of the target chromophores with a high enough photon density to induce the desired reaction. In theory, a single photon can activate a cell, but in actual practice multiple photon absorption is required to achieve the desired degree of reaction.
Phototherapy is athermal and atraumatic, hence achieving selective photothermolysis is of no concern as it would be for surgical or other photothermal applications. On the contrary, penetration of light into living tissue is, however, extremely important in phototherapy, and very frequently displays characteristics which are often in discord with results produced by mathematical models, a point often totally ignored by some researchers. A favorite, but photobiologically false, axiom beloved of phototherapy opponents, is that ‘all light is absorbed within the first millimeter of tissue’. Anyone who has shone a red laser pointer through their finger, transilluminating the entire fingertip and completely visible on the other side, has already disproved that statement. A totally different finding is seen with green or yellow laser pointers, however. Figure 7 is based on a transmission photospectrogram of a human hand captured in vivo over the waveband from 500 nm (visible blue/green) to 1,100 nm in the near infrared.7 The photospectrometer generator was positioned above the hand, delivering a ‘flat spectrum’ of ‘white light’, and the recorder placed beneath it. The wavelength is shown along the x-axis, and the calculated optical density (OD) is on the y-axis, from lower ODs to higher. The higher the OD, the greater is the absorption of incident light, and hence the lower the transmission, or penetration depth into the tissue. It must also be remembered that the OD is not an arithmetic but a logarithmic progression, so that the difference between an OD of 4 and one of 6 is not simply 2, but 2 orders of magnitude, i.e. a factor of 100.
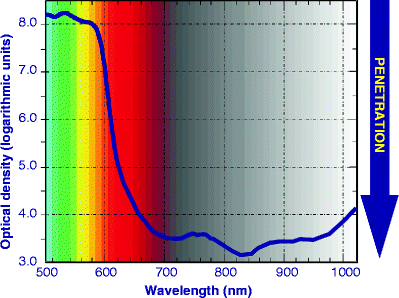
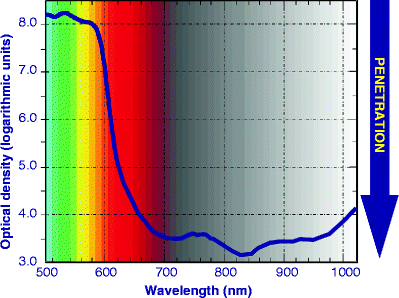
Fig. 7
Photospectrogram of a human hand in vivo. The generator, delivering uniform ‘white light’ at the waveband shown on the x-axis, was placed above the hand and the sensor below the hand. Optical density on the y-axis is shown in logarithmic units. Penetration is shown on the right-hand axis. The further down the curve reaches, the better the penetration at that wavelength into living human tissue (Adapted from Smith7)
From 500 to 595 nm (blue-green to yellow), the OD was from 8.2 to approximately 7.6, respectively, resulting in poor penetration. At 633 nm, the approximate wavelength of the HeNe laser, the photobiological efficacy of which is well recorded, the OD was approximately 4.5. In other words, red light at 633 nm penetrated living human tissue by 3 orders of magnitude better than yellow at 595 nm, because of the pigment-specific absorption characteristics of the two wavelengths. Visible yellow at 595 nm is at the peak of the oxyhemoglobin absorption curve, and is also much higher absorbed in epidermal melanin than 633 nm, which is why the yellow light in the spectrogram did not penetrate at all well into the tissue due to competing chromophores of epidermal melanin and superficial dermal blood. Accordingly, cellular and other targets in the mid to deep reticular dermis are inaccessible to yellow light with sufficient photon intensities to achieve multiple photon absorption in the target cells. The deepest penetration was achieved at 820–840 nm in the near infrared. At this waveband, pigment is not a primary chromophore with proteinous targets as the major chromophore, and this 820–830 nm waveband coincides with the bottom of the water absorption curve. The most successful of the laser diode systems used in laser therapy as distinct to laser surgery, or low level laser therapy (LLLT) as it was also known, delivered a wavelength of 830 nm for this very reason,8 and was shown to penetrate living hands, and even bone, very successfully.9 After around 1,000 nm, water absorption once again starts to play a significant role, and in the curve in Fig. 2 the OD was seen to increase thereafter. In general, shorter visible wavelengths penetrate less than longer visible and near IR wavelengths, up to a given waveband, depending on the absorbing chromophore.
Following these findings, it made a great deal of sense to source LEDs for LED systems at wavelengths already tried, tested and proven in the more than three decades of laser therapy application and research, so LED systems delivering 633 nm or thereabout in the visible red and 830 nm in the near infrared, and at high enough photon densities, have been reported as having significant effects on their target tissues at a good range of depths well into the mid and even the deep reticular dermis. The usefulness of visible red and near IR LED phototherapy has already been reported in a wide range of medical specialties, including dermatology. Yellow light at 590–595 nm has also attracted attention, but the penetration properties of yellow light must be carefully considered, as illustrated in vivo in Fig. 7. From the standpoint of photobiological theory, yellow light has very good potential specificity in a number of subcellular and haematological targets, such as cytochrome-c oxidase, however its poor penetration into the intermediate and deeper dermis, where cellular targets such as fibroblasts lie, limits the practical efficacy of yellow light in living tissue. Blue light at around 415 nm has very interesting properties regarding the eradication of the bacterium Propionibacterium acnes (P. acnes) although the photoreaction is different and will be discussed later in the chapter. LED systems with many other wavelengths have been produced, but basically they have very little or no published work to back up the claims of the manufacturers. ‘Any old LED will not do’ is an axiom which must be borne in mind by the dermatologist wishing to incorporate LED phototherapy into his or her practice.
Finally, the different wavebands, visible light and invisible infrared light, have different primary mechanisms. Absorption of visible light photons at appropriate levels induces a photochemical reaction, and a primary photochemical cascade occurs within the cell, usually instigated by specific components in the respiratory chain of the mitochondria, the adenosine triphosphate-producing power-houses of the cell.10 Infrared photons, on the other hand, are primarily involved in photophysical reactions which occur in the cell membrane, changing the rotational and vibrational characteristics of the membrane molecules. Through subsequent activation of the various membrane-located transport mechanisms, such as the Na++/Ca++ and Na++/K++ pumps and changes in the cell permeability, changes occur in the chemical and osmotic balance in the cytosol, finally resulting in the induction of a secondary chemical cascade which gives more or less the same endpoint as the visible light photons, namely cellular activation or proliferation.11 These photoreactions are illustrated schematically in Fig. 8.
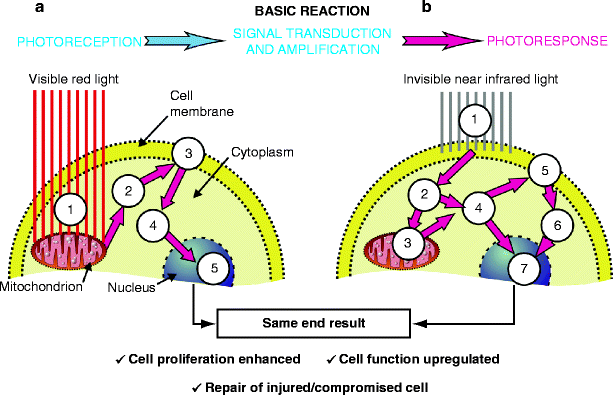
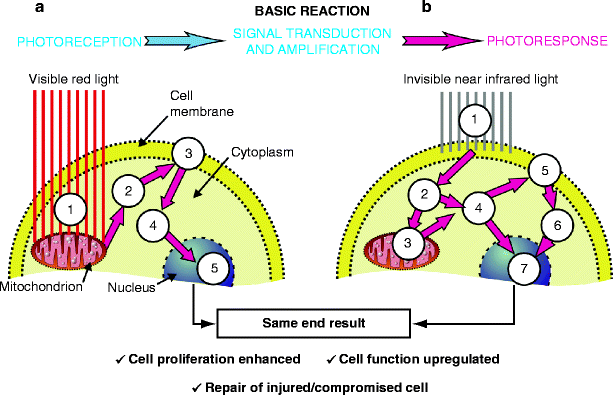
Fig. 8
Schematic depicting photoreception (absorption) of light in a cell, and the subsequent wavelength-specific response. The basic reaction as defined by Karu is absorption, which is followed by signal transduction and amplification within the cytosol, and leads to the photoresponse. (a) (1): Visible red light induces a primary photochemical cascade initiated in the mitochondrion, the energy factory and cell power house, which results in increased levels of nicotinamide adenine dinucleotide (NAD) extremely important in a wide range of redox (reduction-oxidation) reactions, one of the results of which is the generation of adenosine triphosphate (ATP) which is the ‘gasoline’ for the cell. (2): The increased levels of cytoplasmic ATP fuel the membrane transport pumps, the Na++K++ and Ca++K++ pumps (3) which induce extra- and intracellulation of messenger Ca++ ions and protons (H+) which are elementary particles carrying a positive electric charge, the flow of which is used to generate energy from ATP via ATPase. Cytoplasmic levels of Ca++ ions and H+ dramatically increase. (4) This in turn upregulates intracellular signaling including mRNA production from ribosomes on the rough endoplasmic reticulum, and finally (5) nuclear activity is also up regulated. (b) In the case of near infrared light, the primary mechanism of absorption is completely different (1) resulting in a photophysical reaction which changes the energy levels of the cell membrane, in which near IR energy is absorbed. This kick-starts the Na++K++ and Ca++K++ pumps so that cytoplasmic levels of Ca++ and H+ dramatically increase (2) and (4), prompting the mitochondrion to manufacture more ATP to fuel the increased energy requirement (3), thereby raising cytoplasmic levels of ATP (4) which again impacts on the transport mechanisms of the membrane not affected by the near IR light. Despite the totally different pathways, the end result is however the same as in the case of visible light, namely further cyclic increased energy levels in the cytoplasm (6) and upregulation of nuclear activity (7)
To sum up, the wavelength of a therapeutic source therefore has a double importance, namely to ensure absorption of the incident photons in the target chromophores, and to be able to do so at the depths at which these chromophores exist. The waveband in which the wavelength of the incident photons is located determines not only which part of the cell is the target, but also the primary photoaction. Wavelength is thus probably the single most important consideration in LED phototherapy, because without absorption, there can be no reaction.
Photon Density
Light energy travels in the form of photons. It is obvious that the more photons are incident per unit area of tissue, the greater will be the bioeffect. This incident photon intensity is called the power density, or irradiance, of a beam of light. The power density (PD) is an extremely important factor, following wavelength, and is calculated using the following formula:
where OP is the output power incident on the target in watts (W) and TA is the irradiated area in square centimeters (cm2). PD is usually expressed in watts per square centimeter (W/cm2) or milliwatts (mW)/cm2. It is the power density of a beam that will determine more than anything else (apart from wavelength) the magnitude of the bioeffect in the target tissue. Consider Table 2, where a laser with a constant incident output power of 2 W targets tissue with a range of spot sizes. Simply changing the spot size, and thus the power density, can have dramatically different effects on the target tissue. Because the power density is worked out per unit area, calculated by the formula πr 2, where π is the constant pi, 3.142, and r is the radius (half the diameter) of the irradiated area, we have to remember that there is an inverse square ratio between spot size and power density for a constant output power. Doubling the spot size will not cut the power density by one-half, but by one quarter: increasing the spot size by a factor of 10 will cut the power density by one-hundredth, and vice-versa.
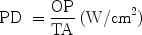
Table 2
Illustration of the importance of altering the power density to achieve a complete range of bioeffects from incision to photobiomodulation with a constant incident output power
Incident power (W) | Spot size (Ø, units as given) | Power density (W/cm2) | Bioeffect |
---|---|---|---|
2 | 100 μm | 25,000 | Incision; excision |
2 | 200 μm | 6,250 | Vaporization; deep coagulation |
2 | 1 mm | 250 | Mild coagulation; protein denaturation |
2 | 1 cm | 2.5 | Athermal, atraumatic photobiomodulation |
In LED phototherapy, it is therefore necessary to achieve a high enough incident photon intensity to achieve the desired degree of multiple absorption in the target cells, but not so high as to cause any degree of photothermally-mediated changes in the tissue architecture, in other words ideal LED phototherapy should achieve athermal and atraumatic photobiomodulation. The Arndt-Schultz law, first appearing in the mid-nineteenth century, states that weak stimuli excite biologic behavior, stronger ones favor it, powerful ones arrest it and very powerful ones retard it. This was adapted by Ohshiro and Calderhead in 1988 into the Arndt-Schultz curve to explain the efficacy of LLLT (Fig. 9)8 ’ 12 from which it is clear that photon intensity should not be too weak (no reaction) or too strong (retardation or cell death) but must be adjusted to achieve maximum optimum photobiomodulation of the target cells or materials.
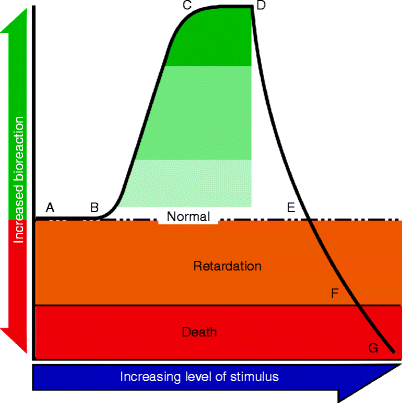
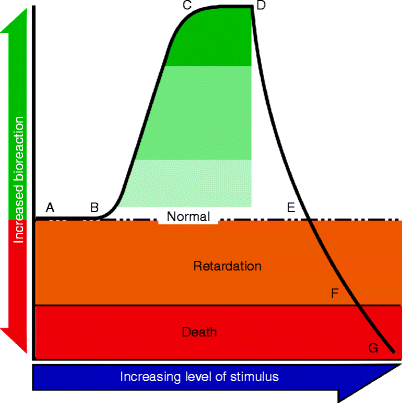
Fig. 9
Ohshiro and Calderhead’s Arndt-Schultz curve (1988, 8), based on the Arnd-Schultz law. From stimulus strength A to B, no reaction occurs: the stimulus is too weak. From B to C there is a sharp rise in bioeffect, plateauing at C-D. This curve is based mostly on incident power density (photon intensity), but the ideal combination of intensity and dose in phototherapy must therefore be attained to reach the effect shown by the dark green shaded area, preferably as much as possible at the C-D effect plateau. From point D onwards there is a sharp drop in the effect, although it is still higher than normal until point E. Strength B-E corresponds to the zone of athermal photobioactivation in Fig. 5 above. At stimulus strength E-F the bioeffect is gradually retarded, corresponding to the protein denaturation/ degradation zones in Fig. 5, and target death results from strength F-G corresponding to the coagulation and vaporization zones in Fig. 5
A final note on intensity: one single LED, even one of the new generation of LEDs, when used on its own, will not achieve anywhere near a clinically useful photon intensity in the target tissue (Fig. 10a). When multiple LED’s are mounted close together in a planar array, however, (cf Fig. 3) and precisely positioned positioned according to the angle of divergence of the beam, the interaction where the beams impact with each other gives an extremely intense photon density due to the phenomenon of interference, particularly with the physical forward- and backward scattering characteristics of red and near IR light which result in the greatest photon intensity being beneath the surface of the skin, exactly where it should be to achieve the optimum therapeutic effect (Fig. 10b). If the distance between the LEDs is too great, however, then the intensity will drop off dramatically because of the lack of interaction between the individual LED beams (Fig. 10c).
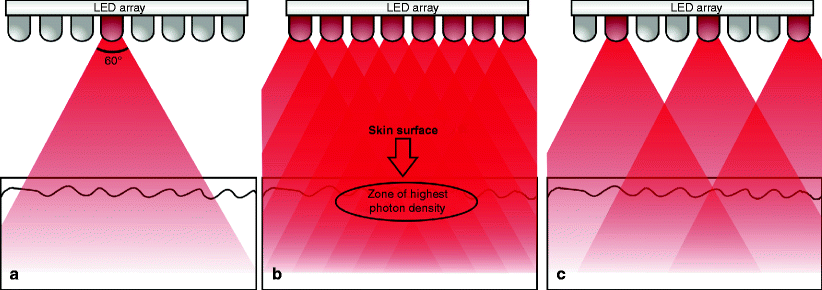
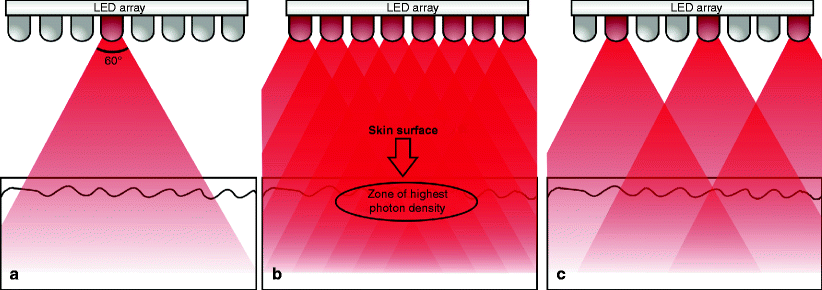
Fig. 10
Arrays of precisely spaced multiple LEDs are required to achieve clinically useful photon densities in tissue. This illustration is modeled on the actual LED array seen in Fig. 3 above, and is to scale. The distance from LEDs to the tissue is approximately 2.5 cm. (a) A single LED has insufficient photon intensity to achieve any recordable clinical effect. (b) On the other hand, when LEDs with similar output characteristics are mounted a precise distance apart to make use of the 60° divergence, the beams will interact where they cross each other to produce an extremely high photon intensity due to the phenomenon of photon interference. When this is coupled with the very strong forward and backward scattering characteristics of red light, which is even stronger for near IR energy, a zone of extremely high photon density, greater even than the intensity at the LEDs themselves, is created under the surface of the target tissue. (c) If LEDs are spaced too far apart, the photon intensity is sacrificed and is not clinically useful. This is the case in treatment heads with individual LEDs of different wavelengths
Dosimetry
So far, the time for which light of a given irradiance or power density is incident on a target has not been mentioned. When treatment time comes into the therapeutic equation it quantifies the dose of light delivered. When 1 W of power is incident on target tissue for 1 s, the energy delivered is 1 J. The joule in itself is a particularly useless therapeutic parameter since it expresses only power over time, and does not take into account the unit area of tissue being treated. The most important parameter for the therapeutic dose in LED phototherapy is the energy density (ED), also known as the spectral fluence. ED is calculated as follows:
where OP is the output power incident on the target in watts, t is the time in seconds and TA is the irradiated area in cm2. ED is expressed in joules/cm2 (J/cm2). However, too much is often made of the dose as the most important parameter in phototherapy, and criticism has been leveled at an LED system that ‘the dose is too high’. In fact, as stated in the previous subsection, it is the power density, rather than the energy density, which more than anything else determines the bioreaction, and this is illustrated in Table 3 where a constant dose of approximately 25 J/cm2 can achieve the entire gamut of bioeffects from pure athermal photobiomodulation to severe photodestruction. The total uselessness of joules as a meaningful parameter is also illustrated: the greatest amount of energy in the table, 2,000 J, produced a phototherapeutic effect, whereas the smallest, 8 mJ (0.008 J), produced a photosurgical effect. In an experiment performed 20 years ago by the author of this chapter. The exposed rat knee joint, both encapsulated and unencapsulated, was irradiated with a GaAlAs diode laser giving an incident power density of 1 W/cm2. A range of doses was applied from 20 J/cm2 (20 s exposure) up to 1,800 J/cm2 (30 min exposure). Tissue was examined macroscopically and microscopically immediately after irradiation for any signs of damage: none was found. The wounds were closed and followed up at different time points over 2 weeks. No differences were seen in coded specimens from each group at all time points regarding morphological changes compared with the unirradiated control specimens. In pharmaceutical science, the medicine must be correct before adjusting the dosage. In phototherapy, the power density is analogous with the medicine, and the energy density is the dose. If the medicine is incorrect, i.e., a photon intensity which is too low (no effect) or too high (photothermal damage) no amount of playing around with the dose will achieve the optimum result.
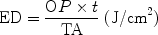
Table 3
This illustrates a variety of bioeffects (Δα) achieved with the same approximate energy density, or dose, of 25 J/cm2. As can be seen from the table, the power density (PD) is the most important determinant of the bioeffect and the energy density given alone is therefore not a real determinant of effect
P | SØ | [a] (cm2) | PD (W/cm2) | t | e | ED (J/cm2) | Δα |
---|---|---|---|---|---|---|---|
100 W | 10.0 cm | 78.6 | 1.3 | 20 s | 2,000 J | 25 | – |
50 W | 3.5 mm | 0.1 | 500 | 100 ms | 5 J | 25 | + |
10 W | 1.0 mm | 0.0008 | 1,250 | 20 ms | 0.2 J | 25 | ++ |
1 W | 200 μm | 0.0003 | 3,180 | 8 ms | 8 mJ | 25 | +++ |
75 mW | 3.0 mm | 0.07 | 1.1 | 23 s | 1.725 J | 25 | – |
Temporal Profile of the Beam
The temporal profile of a beam of light energy simply means the output mode in which the light is delivered to the target. There are two modes, continuous wave (CW) and pulsed. In CW, as the name suggests, when the light source is activated the power reaches its maximum level, from mW up to 100 W or so, and stays there till the system is switched off (Fig. 11a, left pane). An alternative to CW is when the beam is ‘gated’ to produce a train of square waves: this is often incorrectly referred to as ‘pulsed’ light (Fig. 11a, right pane). Gating can be accomplished by a mechanical shutter, or be achieved by simply switching the light source on and off. The correct name for this process is ‘frequency modulation’, because an exogenous frequency (the on-off sequence) is being superimposed on the inherent frequency of the beam which is predetermined by the wavelength, each wavelength having a fixed frequency. For example, near infrared at 830 nm, visible red at 633 nm and visible blue at 415 nm have ‘built in’ frequencies of approximately 3.6 × 108, 4.7 × 108, and 7.2 × 108 MHz. From this it can be seen that as the wavelength decreases, the frequency increases. Increased frequency is also positively associated with an increase in energy of the individual photons, expressed as electron volts (ev), and for the previous three wavelengths the respective photon energies are approximately 1.49, 1.96 and 2.99 ev. Photon energy determines the type of interaction between the incident light and skin cells. For 830 nm, as explained already, photophysical rotational and vibrational changes occur in the electrons making up the cell membrane, whereas for visible light there is a direct induction of an intracellular photochemical cascade. At very high ev values, such as those associated with ultrashort wavelengths, such as X- and γ-radiation, the very large photon energies result in molecular disassociation of cells with sufficient exposure, in other words the cells are literally blown apart.
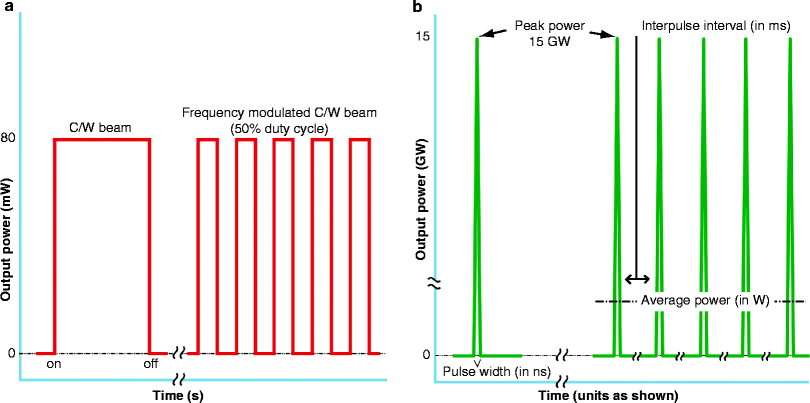
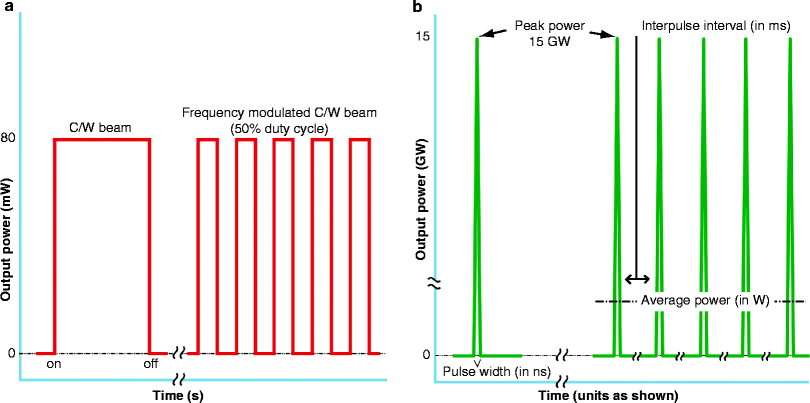
Fig. 11
Temporal profile of a beam of light. There are 2 basic profiles, continuous wave (CW) (a) or pulsed (b). In CW (a, left pane), the system is switched on, the light very rapidly reaches its maximum, and remains there till the system is switched off. This CW beam can be ‘gated’ mechanically or electrically, i.e. rapidly switched on and off (a, right pane), which is often incorrectly referred to a ‘pulsing’. The correct name is frequency modulation. This gives a series of rectangular waveforms: a 50% duty cycle is illustrated. When a laser beam is truly pulsed, a tremendously high peak power, measured as high as gigawatts (GW) is released in an ultrashort pulse interval, measured in nanoseconds (ns) (b, left pane). If a train of these pulses is emitted with a comparatively long interpulse interval of milliseconds (ms) (b, right pane), then the target tissue ‘sees’ only the average power of the beam, measured in watts. This is called quasi-CW, also known as ‘superpulsing’ the beam
In a true pulsed beam, from a high-powered laser, an extremely high peak power is reached in a spike-like waveform, with a very short pulsewidth, 1 ms or even in ns. The peak power may be in mega- or even gigawatts.(Fig. 11b, left pane) If a train of such true pulses is delivered with a set interpulse interval often orders of magnitude longer than the pulse width, then the target tissue ‘sees’ only the average power of the beam, usually at CW output levels. This is also referred to as ‘superpulsing’, or more correctly, quasi-CW (Fig. 11b, right pane). No current LED system is capable of delivering a true pulsed beam.
LED Phototherapy: Mechanisms of Action
LEDs are ideal for photon absorption therapy (PAT), an atraumatic and athermal direct exchange of energy raising the target’s action potential LEDs can be successfully used in photodynamic therapy (PDT) Exogenous PDT with 5-aminolevulinic acid (5-ALA) for treatment of non-melanoma skin cancers and severe photodamage. Endogenous PDT in porphyrins endogenous to Propionibacterium acnes, for example, in the treatment of acne. |
When light energy is incident on a target, the reaction in the target following absorption is known as the mechanism of action. In LED phototherapy, there are two main mechanisms of action: photodynamic therapy (PDT) and photon absorption therapy (PAT), which are totally different mechanisms of action.
Photodynamic Therapy (PDT)
PDT can be exogenous or endogenous, the better known form of which is exogenous.
Exogenous PDT
Exogenous PDT is typically defined as: “The use of a chemical, given orally, intravenously or topically (directly to the skin), that can be activated or energized by light to destroy a target tissue in which the chemical or substance has preferentially located. This activation causes the formation of new molecules and free radicals such as reactive oxygen species (ROS) which may also form other chemicals that, in turn, may destroy the targeted material to a varying extent, such as through ROS-mediated apoptosis of the photosensitized cells or closure of blood vessels feeding the target tissue.” PDT is another arm of phototherapy, and whilst exogenous PDT is thus still an athermal reaction, it is not atraumatic as deliberate induction of apoptotic cell death is the main goal. The first main application for photodynamic therapy was in the treatment of certain cancers, with such photosensitizers as hematoporphyrin derivatives activated with low incident levels of laser light, particularly with visible red light such as from the HeNe laser due to this wavelength’s better penetration than the shorter visible wavelengths in living human tissue.13 This activated an oxygen-dependent phototoxic cytocidal action within the cells containing the agent, and the free radical singlet oxygen (1O2), a short-lived product from the reaction between an excited sensitizer molecule and oxygen, played a very important part in the induction of cell death (apoptosis) and destruction of the microvasculature feeding the tumor.
One of the first applications for LED phototherapy was in fact PDT for non-melanoma skin cancers (NMSCs), such as basal cell carcinomas and superficial squamous cell carcinomas, or severe sun damage such as actinic keratosis with the use of another exogenously-applied compound, 5-aminolevulinic acid or 5-ALA in any of its forms. This application continues to the present with good success and robust long-lasting results.14 ’ 15 The topically applied 5-ALA penetrates into the dermis under an occlusive wrap, and is converted as part of the mitochondrial-based heme cycle into coproporphyrin III (Cp III), a member of the powerful porphyrin photosensitizing family. When the maximum amount of Cp III has been converted, the remainder of the 5-ALA is converted into another porphyrin, protoporphyrin IX (Pp IX). These two porphyrins become the specific targets of the LED energy at specific wavelengths, and, following photoactivation, nonselectively damage all of the superficial dermal tissue in which they exist, as illustrated schematically in Fig. 12.
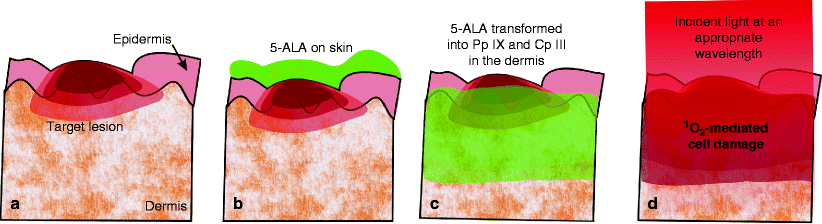
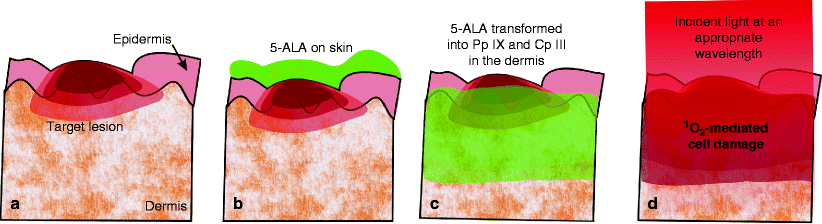
Fig. 12
Nonselective en bloc infiltration of skin by Pp IX and Cp III of 5-ALA origin illustrated schematically. (a) Target lesion in superficial dermis. (b) 5-ALA ointment applied topically to epidermis. (c) As 5-ALA penetrates en bloc into skin cells, it is transformed into Cp III and Pp IX, photosensitizing porphyrins. (d) Light at an appropriate wavelength activates the porphyrins to produce powerful but very short-acting reactive oxygen species, such as singlet oxygen, and the affected skin cells die through oxidative-stress mediated apoptosis (induced cellular destruction)
When a photoreaction is desired such as in 5-ALA PDT for any purpose, an action spectrum has to be run to investigate the action potential of a range of wavelengths in the target compound. Figure 13 shows the absorption spectra of Pp IX and Cp III. There is a very large peak at 415 nm in the visible blue Soret band, but as will be remembered from the previous section on wavelength, blue light has very poor penetrative capability into the dermis, and so it would not cause deep enough damage to treat NMSCs successfully. Another much smaller peak is however seen at around 633 nm, which was used in the early days of hematoporphyrin derivative PDT for other cancer types as a much better-penetrating wavelength, thus giving a much deeper zone of porphyrin activation and hence a deeper zone and greater volume of controlled photodamage. Red 633 nm LED-activated 5-ALA has been successfully used for NMLCs and actinic keratoses, photorejuvenation and inflammatory acne vulgaris. These will be discussed in more detail in the appropriate subsection on LED phototherapy in clinical practice.
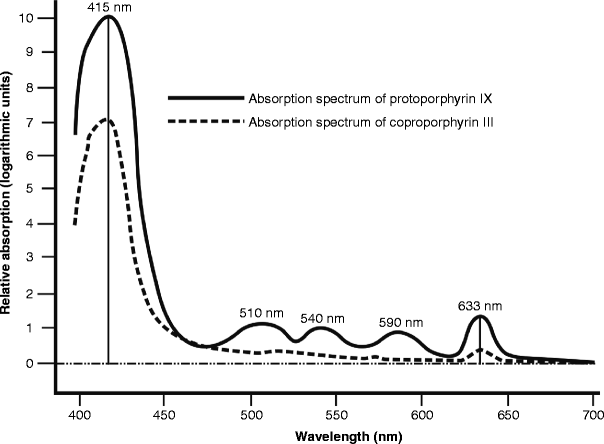
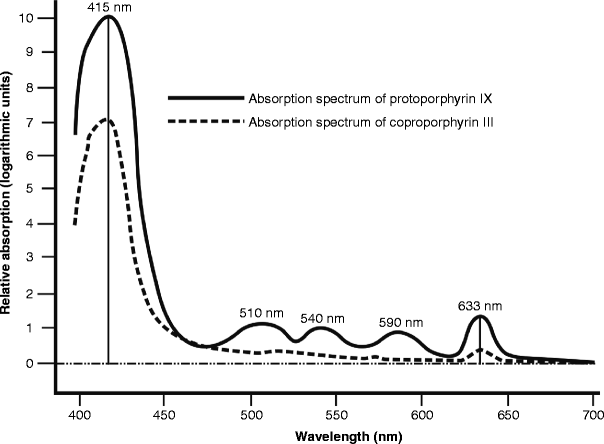
Fig. 13
Action spectra for coproporphyrin III and protoporphyrin IX. Note the extremely high peak at 415 nm, and the minor peak at 633 nm, visible red, particularly in Pp IX
Endogenous PDT
Exogenous PDT as discussed above depends on an external photosensitizer, such as 5-ALA. In endogenous PDT, the photosensitizer, or photosensitizing substances, can be found occurring naturally within the target cells or tissue. The exogenous application of 5-ALA induces the synthesis of the porphyrins Pp IX and CP III nonselectively in the epidermis and dermis under the area of application as already explained above. However, in the case of acne vulgaris the inflammatory acne lesions are associated with the presence of their causative bacterium, Propionibacterium acnes (P. acnes). It has been well demonstrated that both Pp IX and Cp III are endogenous to active P. acnes, and the more active is the bacterium, the higher the porphyrin concentration.16–18 Referring again to Fig. 13, maximum photoactivation of both Pp IX and Cp III occurs at around 415 nm. Light at that wavelength, with a high enough photon intensity, could therefore achieve activation of the porphyrins within the P. acnes, thereby selectively destroying or at least severely damaging the P. acnes through oxidative stress-induced apoptosis,19 but without harming the surrounding skin cells. Endogenous PDT could therefore be applied in the light-only treatment of inflammatory P. acnes lesions without the need for any exogenous 5-ALA. This will be discussed in more detail in the appropriate part of the following section.
Photon Absorption Therapy (PAT)
Basically the majority of the information in subsections 1 and 2 has been based on the concept of photon absorption therapy
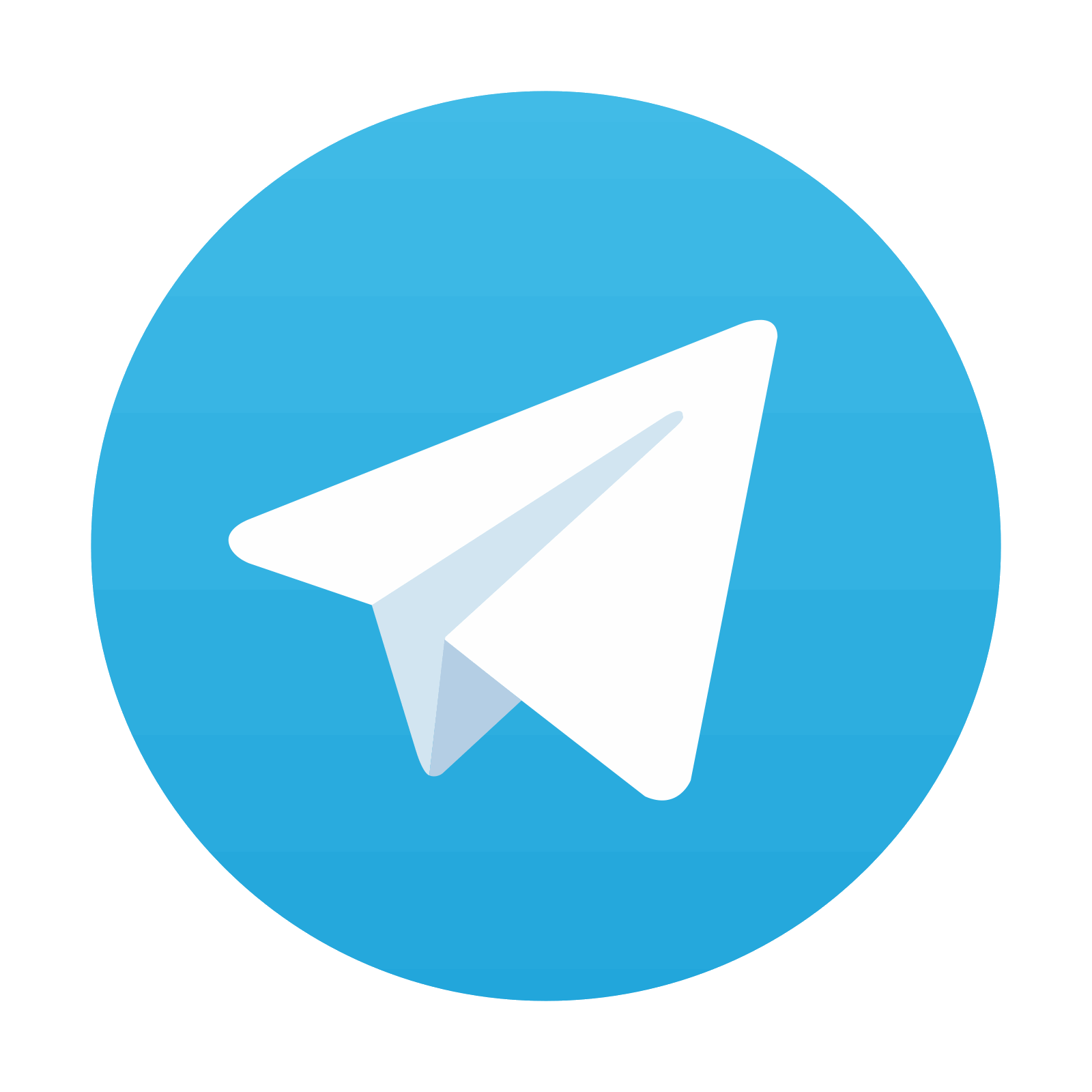
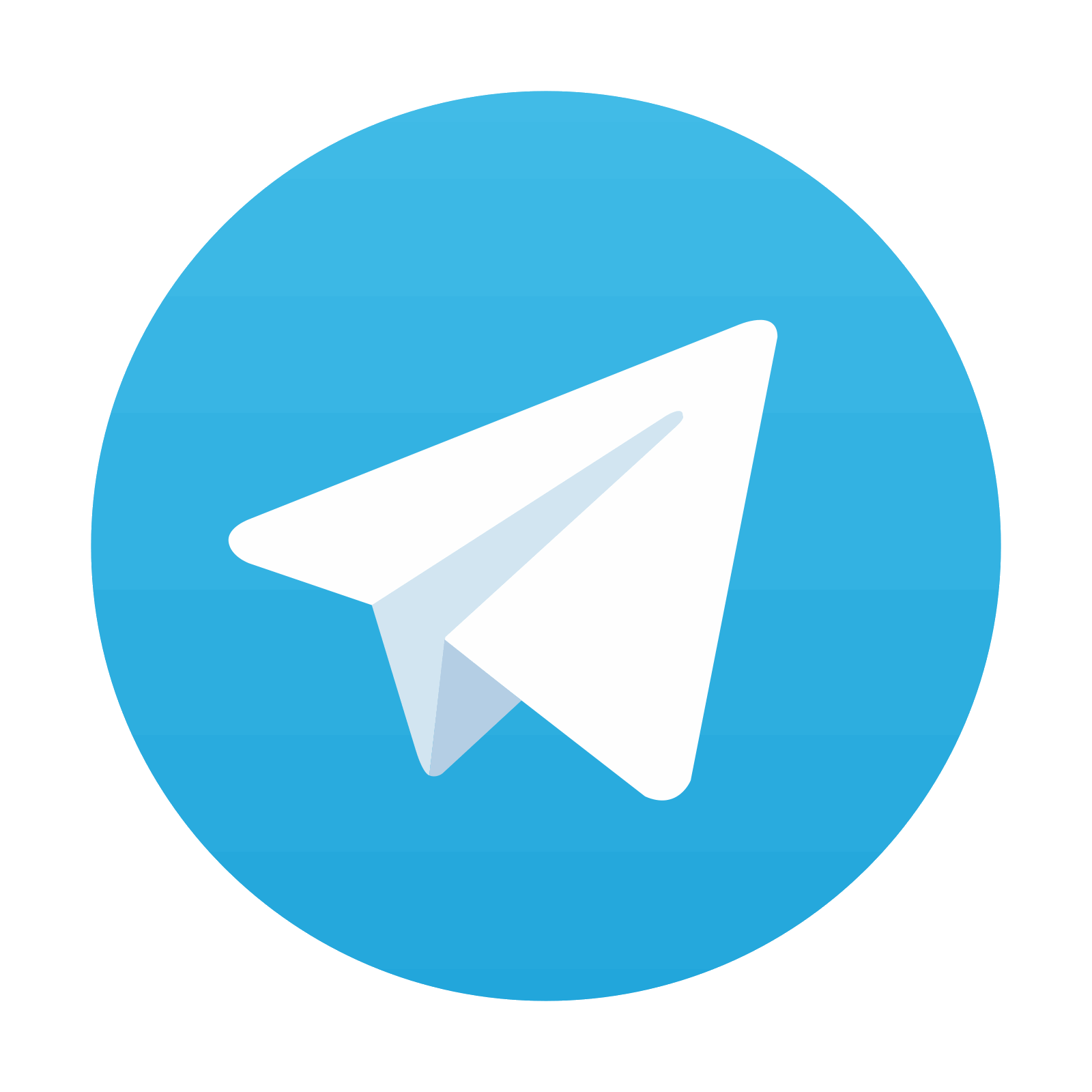
Stay updated, free articles. Join our Telegram channel
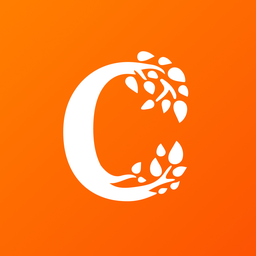
Full access? Get Clinical Tree
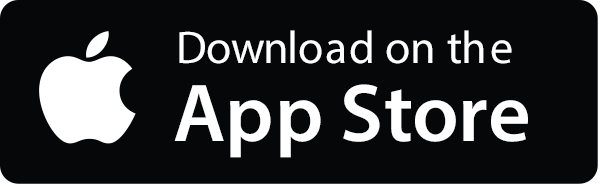
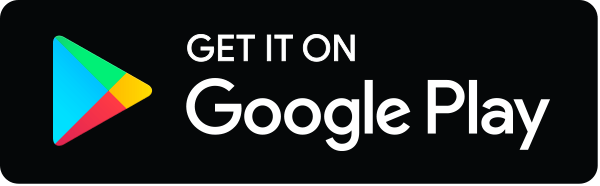