Fig. 1.1
Morphological differences between capillary beds of various tissues. Confocal micrographs of blood vessels, shown in green, found in the retina, inner and outer choroid, skin, brain, heart, liver and skeletal muscle of adult transgenic Tg(fli:EGFP)y1 zebrafish, expressing enhanced green fluorescent protein (EGFP) in endothelial cells. The white size bar indicate 100 μm

Fig. 1.2
Vascular endowment is balanced by the metabolic needs of the tissue. In healthy tissue the vessel density and perfusion (red balls) is balanced according to the metabolic activity of the tissue (blue balls; left image). Should the metabolism increase (middle image), perfusion and oxygen delivery will also increase through vessel dilation, angiogenesis and erythropoiesis. Is however the vascular density or perfusion higher than the needs of the tissue (right image), excessive vessels will regress or the metabolic needs of the tissue will increase (phenotypic switch). In diseased tissues, excessive vessels do not regress but rather sustain pathological transformation or growth of the tissue
Blood vessels, however, are not only a conduit for transport of oxygen, nutrients and metabolic waste products. Many factors important for organizing the functions of various organs to act in concert with each other (i.e. endocrine regulators) also use the vasculature as a route of communication and the vessels play an active role in the signaling process. For example, insulin-dependent and in-dependent glucose uptake by cells in the brain, where there is no passive leakage of glucose across the capillary, is mediated by active transport of glucose across the wall of the blood vessels by Glut1 and Glut4 glucose transporters [47, 48]. Furthermore, hormone receptors are expressed at high levels in the vessels which, in response to hormone stimulation, produce cytokines that mediate some of these hormone-dependent effects on the tissue, thus playing very important roles in endocrine regulation [49, 50]. Also under pathological conditions such as tissue inflammation, the tissue recruit immune cells to fight the inflammatory stimulus (often invaded bacteria) by activating the vessels locally, an activation that lead to the presentation of molecules including ICAM and VCAM, on the inner surface of the vessel which are recognized by the immune cells and mediate their movement across the vessel and into the tissue specifically at the site of inflammation [49]. These functions are also important for the physiologic trafficking of immune cells to various lymphoid tissues in the organism [51].
The vasculature not only transports chemicals and cells, it is also critical for the thermoregulation by transporting heat [52]. Brown adipose tissue or skeletal muscles are the main sources of heat generation due to their high expression of uncoupling proteins and inherent storage of energy either as fat or glycogen. From here, warm blood is pumped for example through the skin, which is a main tissue responsible for heat/cold sensing [53]. The important role of the blood for maintaining temperature is mirrored in the fact that core body temperature decreases during the inactive period (i.e. night in humans), when the heat generating muscle and brown adipose tissues are less active [54], although many of the other tissues exhibit similar metabolic activity.
Locally, in the tissues, blood vessels also provide key signals for the surrounding cells which are crucial for the specific function. Especially un-differentiated stem- and progenitor cells are known to exist predominantly in so-called vascular nieches, in which these cells make direct contact to endothelial cells, contacts that are crucial for maintaining the undifferentiated state of the cells [55, 56]. As such in tissues as different as the intestine, bone marrow, skin, brain and in tumors, the non-differentiated stem/progenitor cells are specifically found associated with the vasculature [57]. Also other, differentiated cells types require contact with vascular endothelial cells in order to maintain their phenotype. An example of this has recently been demonstrated in adipose tissue, where pericytes loose their identity and transform into adipocytes when their contacts with endothelial cells are disrupted [58]. Similarly, vessels-associated macrophages are primarily of the alternatively differentiated type, whereas non-vessel associated macrophages to a higher extent exhibit the classically activated phenotype [59] indicating that vessel contact may induce or maintain processes required for alternative differentiation and associated functions of macrophages.
During development, the vasculature provide key signals required for organogenesis, regulation of organ size and shape as well as differentiation and specification of cell types in the various organs. The development of the alveoli in the lungs, for example, depends on signals provided by the developing lung vasculature [60]. Should blood vessel growth be inhibited during lung development, this will lead to a failure in the development of alveoli. Also in the kidney, the vasculature provide crucial signals required for differentiation and formation of the glomeruli [61] and in the liver endothelial cells may be important for both the early development of the fetal liver, the structural development of the liver lobules and for the growth and size of the liver both during development and during regeneration [62]. Such regulatory roles of blood vessels also dominate in the regeneration of other organs and tissues, including the skin, in adults [63]. Fin regeneration in fish, for example, is stunted if blood vessel growth is inhibited leading to incomplete regeneration and a smaller fin compared to the size prior to amputation [64]. It is likely that blood vessels do more than simply provide oxygen and nutrients to the developing tissue and as such regulate its size. In zebrafish embryos, oxygenation is not dependent on perfusion during early development as the small size of the embryos allows for passive diffusion of oxygen through the tissues as the main mechanism of oxygen delivery [19]. Yet, the development of for example the kidney and liver still requires blood vessels [65, 66] indicating that there may be some still poorly characterized signals being produced by the vessels which are important for organ development.
In order to accomplish all the functions and respond appropriately to systemic or tissue-specific regulatory signals, blood vessels combine several different cell types tasked with specific regulatory properties that work together in one functional unit. Blood vessels are subdivided in three general classifications based on the direction of blood flow, pressure, vessel structure and blood oxygenation level; arteries carry high-pressure, highly oxygenated blood in an efferent manner to the tissues, capillaries are small caliber vessels that distribute the blood within and actively communicate with the tissues as described above, and veins are large caliber vessels that drain the blood from the tissues and transport it in an afferent manner back to the heart. Arteries, capillaries and veins are anatomically highly different and are also regulated differently. Anatomically, the wall of large arteries and veins may be sub-classified in at least three different regulatory domains known as the intima, media and adventitia (Fig. 1.3). The innermost intima consists of endothelial cells, peri-vascular cells known collectively as pericytes and their shared basement membrane constructed from collagenIV, laminin, nidogen, and perlecan/proteoglycans [67]. The media (or tunica media) is a smooth muscle cell layer that covers the intima and particularly for large arteries can be many cell-layers thick and constitute the largest proportion of the vessel. Veins have thin media as the diameter of veins is to a larger extent regulated passively by the amount of drained blood rather than actively via smooth muscle contraction/dilation. The adventitia consisting of fibroblasts, connective tissue, nerves and for the largest vessels even its own vasculature (vasa vasorum) constitutes the external barrier of the vessel and is therefore the toughest part of the wall. In large arteries such as the aorta it is elastic due to the presence of elastin, which is required for the buffering of the large changes in blood pressure as the blood is pumped out of the heart. Capillaries contain only a primitive intima and generally have no media or adventitia (Fig. 1.3). Arteries and veins of different organs are rather similar in structure, function and regulation. Capillaries, however, communicate with the tissue in which they reside and are as such highly diverse depending on the needs of the tissue. For example, capillaries in the liver, glomeruli and endocrine organs such as the adrenal gland, pancreas and thyroid are very leaky due to the existence of small holes (fenesters) in the endothelium allowing small compounds and sometimes even cells to pass freely between the tissue and the vasculature [68]. In the skeletal and cardiac muscle, the capillaries are straight, and with little branching, which allow for very efficient perfusion and in the brain the capillary wall is covered with numerous other cell types including pericytes, astrocyte end-feet and even nerve endings, a structure known as the neurovascular unit or the blood-brain barrier. Also the expression profiles of the endothelial cells are different depending on the tissue, reflecting these differences in structure and function. As such, the endothelial cells of the capillaries are unique in each tissue, mirroring the specific functions of the tissue and the associated requirements for communicating with the rest of the organism via the circulation. Recently it was discovered that also the pericytes are not a homogeneous population of cells but rather that various different types of pericytes are present in the vessels and that the composition is different between organs [69, 70]. In addition, other cells such as stem/progenitor cells, immune cells and possibly other cell types may also constitute part of the wall of capillaries or larger vessels. The role of pericytes and other peri-vascular (also known as mural) cell types are poorly understood beyond that they are important for vessel stabilization and survival, but are likely important for tissue homeostasis as well as involved in pathophysiological conditions such as fibrosis, regeneration and inflammation. Possibly, some of these functions may arise from their crosstalk with endothelial cells [71], endowing the highly diversified anatomy and functions found in the capillary beds throughout the organism and as such they may be key players in tissue-specific regulation of the vasculature.


Fig. 1.3
Vascular anatomy. The anatomy of the vessel wall in large arteries, veins and capillaries are illustrated in the top row. The vessel wall consists of the intima (endothelial cells, pericytes and basement membrane), media (smooth muscle cells) and adventitia (fibroblasts, nerves and capillaries). Arteries have a thicker media and more nerves and vessels in the adventitia compared to veins. Capillaries lack media and adventitia. Capillaries in different tissues exhibit widely different morphologies as illustrated in the lower row. Capillaries in the liver (and other endocrine organs) are fenetrated and leaky, whereas a subset of capillaries in the skin lack pericyte coverage and capillaries in the brain and retina exhibit very tight and complex regulation at the vessel wall including coverage with astrocyte end-feet, giving rise to the blood-brain barrier
Hypoxia is the key signal for increasing perfusion to tissues. Hypoxia act via in principle three different routes; the immediate reaction is the activation of endothelial nitric oxide synthase (eNOS) activity, leading to production of nitric oxide (NO) which is one of the most potent signals for relaxing smooth muscle cells and thus dilation of arteries, via activation guanulyl cyclase-mediated cGMP production and down-stream signaling in smooth muscle cells, providing an increased amount of oxygenated arterial blood to flow into the hypoxic tissue [72, 73]. Hypoxia also lead to the increased production of erythrocytes via up-regulation of EPO in the kidneys, as well as blood vessel growth via production of vascular endothelial growth factor locally in tissues (more on this in section “Modes of Blood Vessel Formation and Regression”). This regulation is exploited pharmacologically as patients with high blood pressure are often prescribed nitroglycerin, a potent NO-donor, which will lead to vascular dilation and reduced blood pressure. In addition to direct regulation by hypoxia, blood vessels and in particular arteries are highly innervated and thus regulated by the sympathetic nervous system. As such, perfusion of muscles is enhanced under stressful situations as a part of the fight or flight response, the intestines exhibit increased perfusion shortly after meal intake, and the skin is perfused to a higher degree during exercise or in very warm or cold environments. These responses are in large part due to beta-adrenergic signaling in the neuro-vascular synapses [74]. A third important regulator of vascular function is inflammatory signaling, as sites of inflammation are hyperperfused and leaky, which is important for appropriately mounting the inflammatory defense system. In such instances, cyclooxygenase- or lipoxygenase-mediated production of prostaglandins, leucotrienes or thromboxanes, which have strong vasoactive effects, play a major role in the inflammatory vascular responses [75]. Certain neuropeptides including neuropeptide Y, vasoactive intestinal peptide, melanocyte stimulating hormone and calcitonin gene-related peptide are also important modulators of vascular function, in particular in allergies or asthma [76], in the physiologic regulation of intestinal or central nervous system perfusion. Most of these molecules act on arteries, but will lead to increased blood flow through capillaries which will activate shear-stress sensitive signaling in both down-stream arteries, pre-capillary arterioles and capillary endothelial cells. Such signaling will first and foremost lead to remodeling of the vasculature including circumferential vascular growth, vascular survival (non-perfused vessels regress over time in healthy tissues), leakage and recruitment of peri-vascular cells [77]. Shear-stress signaling however, may also lead to vascular expansion by other mechanisms, including angiogenesis, should the stimulation be sufficient to reach the pro-angiogenic threshold [78, 79], as a mechanism of providing more capillaries to absorb the increased inflow of blood into the tissue and as such reduce the pressure in the individual capillaries. The mechanisms involved in angiogenic expansion of the vasculature are the topic of the next section.
Modes of Blood Vessel Formation and Regression
Blood vessel formation can in principle be divided in two main categories – formation of new vessels by aggregation and vascular morphogenesis of endothelial cells, a process known as vasculogenesis, and the expansion of existing vasculatures, a process known as angiogenesis.
Prior to vasculogenesis, endothelial cells are specified from the hemangioblast progenitor population in the lateral plate mesoderm and migrate during early somitogenesis medially to form a main central endothelial chord [80, 81]. This process is stimulated by sonic hedgehog-mediated vascular endothelial growth factor (VEGF)-A production by the cells in the myotome and thus occurs in a central-to-rostro/caudal fashion. At this point, the endothelial cells are progenitors lacing luminal and basolateral polarization, but as they assemble and form the first vascular chord, they start to accumulate water-filled vesicles in a highly coordinated fashion in which such vesicles in neighboring cells line up with each other in a common plane. These vesicles fuse, both within each cell as well as between adjacent endothelial cells, forming the lumen of the first vessel, the dorsal aorta [82]. An alternative mechanism involving electrostatic repulsion between endothelial cells at the luminal side as a chord-hollowing mechanism has also been described [83]. Once a lumen has been formed, endothelial cells polarize and establish different functionality at the luminal versus the basolateral side, similar to the polarization of epithelial cells. The early vasculature is the site of initial hematopoiesis, where erythrocytes bud off the endothelium and are deposited as the first circulating cells in the blood islands of the yolk sac and the lumen of the aorta [84]. Concurrently, the ventral cells of the aorta also start migrating ventrally, a process that is independent of VEGF-A but instead mediated by repulsive signals between EphrinB2 expressed in the aorta and EphB4 expressed in the migrating cells [85]. These cells eventually coalesce and organize themselves with their luminal side facing inward into a large caliber vessel; the cardinal vein. These two vessels, the dorsal aorta and the cardinal vein connect to the heart and fuse to form a loop at the posterior pole at late somitogenesis stages, thus completing the primitive circulatory loop [81]. Similar processes occurs in the brain where endothelial cells arise in other mesodermal progenitor pools, migrate and coalesce to form the first cerebral arteries and veins [86]. The primitive vasculature expands by sprouting angiogenesis. The first dorsal, arterial sprouts arise at the dorsal aspect of the dorsal aorta and grow as intersegmental arteries, dorsally between the somites in a VEGF-A dependent manner [19], around the same time where the cardinal vein cells migrate ventrally. This is the first example of sprouting angiogenesis in the organism, but vascular expansion of other tissues later in development occurs by largely identical mechanisms. As such, sprouting angiogenesis is responsible for forming the vast majority of all vessels in the organism.
Sprouting angiogenesis can in principle be divided in several individual processes. As the process begins from an already perfused, and often mature, vessel, the first step is to destabilize the vessel wall by local breakdown of the basolateral basement membrane. This is achieved by induction of matrix metalloproteinases (MMPs) including MMP2 and MMP9, as well as other extra-cellular matrix (ECM) proteases [87]. In the case of vessels highly covered by pericytes, these then detach from the endothelial cells, allowing the formation of basolateral pseudopodia in the responding endothelial cells. This is followed by the induction of a tip-cell, i.e. the primary responding endothelial cell which on one hand develops large pseudopodia (filopodia and lammelipodia) allowing the cells to migrate into the tissue and on the other hand suppress similar activation of neighboring endothelial cells through the Dll4-Notch1/4 axis [88–90]. The tip cell then migrates into the tissue, scanning the path with highly dynamic filopodia as it moves and remain intimately connected to its neighboring cells which form the subsequent stalk cells. Stalk cells proliferate and as such extend the connection with the mother vessels as the tip cell drags the new vessel sprout longer into the tissue [18]. Recently, this over-simplified scheme however was revised as it was found that tip and stalk cells compete for the tip cell formation as stalk cells may move to the tip cell position and vice versa during sprout elongation [91]. As such, stalk cells may move relative to each other, but only the tip cell decide the direction of growth for the entire new vessel. When the tip cell meets with another endothelial cell, they fuse and as such terminate the growth of the vessel. This process is called anastomosis and is critical for establishing perfusion [92]. It has been suggested that this anastomosis process is regulated by macrophages which attract tip cells and mediate their fusion [93]. Following anastomosis, the two endothelial cells meeting each other form new tight junctions, which mature, remodel and modify the shape and relative position of the endothelial cells in the previously growing vessels such that their lumens are merged and blood can move between the vessels [92]. The new vessels mature by recruiting pericytes and constructing a new basement membrane which provide stability and survival signals to the vessel. These processes are however not serially induced, vessels mature as they grow and only the distal piece of the sprout is immature. Tip cells constantly secrete platelet-derived growth factor (PDGF)-B, a potent chemoattractant and growth factor for pericytes, which secure the pericyte investment of the vessel as it grows [94]. Pericytes and the endothelial cells themselves also secrete all the factors found in the basement membrane. Angiogenesis is kept in check not only by the initial restriction of sprouting by activated Notch-signaling but also during sprout/vessel elongation as the stalk cells secrete soluble VEGF receptor (VEGFR)-1, which act as a molecular trap on the sides of the vessel such that all the growth stimulatory signals are received only by the tip cells in the very front [95].
High frequency actin turn-over is important for the tip-cell function as they need to rapidly send out and retract filametous-actin rich filopodia while at the same time moving the cell forward through lammelipodia [96]. As such, small rho-GTPases including RhoA, Cdc42 and Rac which mediate the polymerization of actin, cofilin and GTPase-activating proteins (GAPs) which mediate the depolymerization of actin and Arp2/3, wasp and focal-adhesion components which mediate the structural arrangement of the actin cytoskeleton and its connection with the extracellular matrix are all critical elements necessary for sprouting angiogenesis [96]. The filopodia of the tip cells are rich in so-called patterning molecules, receptors for membrane bound ligands presented by the cells in the surrounding tissue that in the most cases exert repulsive signals on the filopodium leading to its regression. These patterning molecules in this way make sure that the vessel is not growing into tissues where it should not, but stay on its intended course [97]. This is particularly clear during development as the intersegmental vessels have a highly stereotypical shape mediated by the inter-somitic boundaries, and never grow into the somites themselves. However, in the case of animals in which semaphoring ligands or plexin receptors have been rendered non-functional genetically, vessels readily grow into the somites and the vascular development is chaotic and ineffective leading to the early embryonic demise of the animal [98]. Interestingly these patterning factors are shared by the axonal growth cones and also important for nerve pathfinding during development [97].
In the stalk cells, restraining sprouting, maintaining cell-cell junctions and proliferation are key for the extension of the vessel and maintaining its connection to the patent vasculature. Many of these aspects are regulated by shear stress as the stalk cells are carrying blood (although perfusion is poor until after anastomosis) and are thus flow-stimulated [99]. The stalk cells also express or respond to “patterning factors” such as notch and jagged but these are instead crucial for mediating the crosstalk between endothelial cells and pericytes necessary for vascular maturation. Other important factors mediating the crosstalk between endothelial cells and pericytes include angiopoietin 1 and 2, and their ligands Tie1/2 as well as transforming growth factor (TGF)beta and the TGFb receptors [100].
In addition to sprouting angiogenesis blood vessels may also grow by splitting themselves in two, a process known as intussusception. Intussusception is characterized by the local luminal bulging of the endothelium proceeding until bulges from the two opposing sides reach each other, forming a trans-vascular hole or “intussusceptive pillar”, which in turn is stabilized by perivascular cells, mainly fibroblasts, moving into and filling the pillar [101]. It has been suggested that this process is primarily induced by VEGF-signaling [102], but may also be mediated by reduced notch-signaling in the endothelium, as it is the case for sprouting angiogenesis [103]. Intussusception, however, involves stalk cells rather than tip cells and the “sprouts” forming the intussusceptive pillar form on the luminal, rather than the basolateral side of the endothelium. As intussusception often lead to the formation of a loop that support circulation, this is considered a way for the endothelium to survive by enabling pro-survival shear-stress signaling under conditions of inhibited survival or growth factor signaling such as pharmacological inhibition of VEGF [104]. Some tissues, such as the lungs and the choroid in the back of the eye, however, have adopted this mode of angiogenic expansion during development [105, 106] indicating that it is also important in physiology. The intracellular processes taking place in the endothelial cells during intussusception is however poorly understood at present.
A variation of intussusception is the recently described pulling of vessels into a non- or de-vascularized area from the surrounding vascularized tissue mediated by myofibroblasts, a process that often precedes sprouting angiogenesis and is, like intussusception, dependent on myofibroblast activity [107]. This is for example seen during inflammation-induced corneal neovascularization or wound healing in the skin, where immediate vascularization of the inflamed cornea or regenerating skin is accomplished by in this way recruiting already existing vessels rather than waiting for new ones to grow [107]. It is however not known if this process plays a role during physiological neovascularization during development.
Large blood vessels are highly stable, but capillaries are constantly remodeling especially in dynamic tissues such as muscle, adipose or liver. Also during development and in wound healing/regeneration the vasculature develops as an overly dense network which then remodels to achieve its final density reflected by the needs of the tissue [108, 109]. As such, hyperoxia or hypoperfusion in blood vessels start a process of vascular regression which can largely be considered as reverse sprouting – the cell-cell junctions between two endothelial cells remodel, the lumen collapses and the junctions are dissolved leading to two tip cells being pulled backward toward the used and stabilizing vessels to which the regressing vessels connect [110]. Alternatively, in pathological situations such as in cancer or neovascular eye diseases, immature pathological vessels may also regress simply by removal of the pro-angiogenic signal, also leaving a few but much more mature vessels that are stabilized and remain in the absence of growth factor signaling [104, 111]. This ordered regression is required for avoiding hemorrhage or leakage of blood or plasma into the tissue. During regression, however, the receding vessels leave the basement membrane behind as empty sleeves, or tunnels in the tissue. Such acellular “ghost” vessels which do not carry blood, but can still be seen by immunostaining for basement membrane markers such as collagenIV, or by other types of imaging techniques, are thought to be a highway for extremely rapid neoangiogenesis should the vessel growth signal emerge again for example during breaks in the anti-angiogenesis treatment procedure or due to recurrence of an inflammatory signal [112]. It is currently not known how this last part of the vessel, the basement membrane, is removed during developmental vascular remodeling or whether such processes could be exploited to generate more complete and long-lasting anti-angiogenic treatments in the future.
Differences in Healthy and Pathological Angiogenesis
The above description primarily pertains, in principle, to both developmental angiogenesis, constructive angiogenesis as a part of tissue regeneration as well as pathological, non-constructive angiogenesis. In pathological conditions, however, the process is deregulated in various ways, leading to formation of chaotic vasculatures that are poorly functional, i.e. tissue perfusion is often not improved, or even decreased, despite increased vascular density [113]. This leads to persistent hypoxia and further hypoxia-induced pathological angiogenesis, thus constituting a vicious circle which aggravates pathological phenotypes [114]. As such in diseases characterized by pathological angiogenesis including chronic inflammatory disorders, cancer or hypoxia-associated retinopathies such as age-related macular degeneration, diabetic retinopathy and retinopathy of prematurity, correcting the vascular function by reducing the pro-angiogenic signaling in the microenvironment for example by using VEGF-targeting drugs are in many cases effective treatments which increase the healthy functions of the inflamed or hypoxic tissue and inhibit disease progression.
A key phenotype of pathological vessels is the chaotic structure which arises from the lack of patterning signals. Many patterning factors are primarily expressed during development and are thus lacking in quiescent adult tissues [115]. While growing endothelial tip-cells re-express vascular patterning receptors, pathological tissues do not express the ligands, or the ligands are proteolytically cleaved to fragments of opposite function compared to the native molecule [116] and thus physiological vascular repulsion does not occur and vessels grow in any direction and do not follow defined trails. Another class of patterning receptor-ligand pairs, namely the semaphoring-plexin system is also involved in pathological angiogenesis, but the regulation is complicated by the fact that these protein families are rather large and different receptor-ligand interactions may exhibit opposing functions on the vasculature [117]. Nevertheless, de-regulation of the expression of patterning factors is an important, yet understudied mechanism for driving the pathological phenotypes of vessels found in inflamed and/or malignant tissues.
Under pathologic conditions, the production and secretion of angiogenic factors occurs in a deregulated manner which contributes to the disorganized overgrowth of vessels. As angiogenic stimuli are often sensed from all directions, the vessels are dragged everywhere at the same time. This is in stark contrast to the spatiotemporal production of for example pro-angiogenic VEGF in the myotome at 24 h post fertilization in zebrafish embryos, which exactly coincide with the sprouting and growth of intersegmental arteries dorsally, towards the myotomes and the subsequent repression of VEGF-A in myotome, when the vessels arrive at this location [19]. The VEGF-production is then rather shifted to the dorsal roof of the intersegmental vascular bed, leading to continued dorsal growth and eventually the formation of the dorsal anastomosing longitudinal vessel (Fig. 1.4). In pathological situations such as in cancer, the pro-angiogenic signals are not turned off or repositioned in a regulated manner, leading to vessels growing into the tissue producing the angiogenic factors and there, grow in all directions, anastomose prematurely, and immediately re-initiate sprouting in a continuous cycle leading to the formation of a vasculature characterized by highly ineffective circulation.


Fig. 1.4
Spatiotemporal expression of VEGF-A drives vascular patterning in zebrafish embryos. In situ hybridization showing Vegfaa mRNA expression in zebrafish embryos at 24–48 hours post fertilization (hpf) is shown in purple in the top row. Vegfaa is initially expressed in the myotomes but gradually relocate to the dorsal roof and ventral floor of the trunk at 36 hpf. At 48 hpf expression emerges in the notochord. These changes in expression correlate with areas of ongoing angiogenesis as shown in the lower row. Blood vessels of Tg(fli1a:EGFP)y1 zebrafish embryos shown in green start sprouting towards the VEGF-A signal at 24 h. At 36 h when Vegfaa expression has shifted to the dorsal roof of the trunk, formation of new sprouts leading to the construction of the dorsal-lateral anastomosing vessel in this site, takes place. At 48 hpf when notochord-expression is initiated, lymphatic progenitors sprout and form the transient parachordal lymphatic vessels. The white or black size bars indicate 100 μm
Persistent angiogenic stimulation also leads to defective pruning/regression of surplus or under-used vessels, a key feature of healthy vascular remodeling. Indeed, the vasculature of pathological tissues can sometimes resemble that of healthy or regenerated tissue prior to the onset of vascular remodeling in terms of containing vessels that are either non-perfused or contribute to inefficient perfusion through futile loops. High levels of proteases which are common to tissues exhibiting pathological angiogenesis prevent vascular maturation by inhibiting the formation of a continuous basement membrane and an appropriate recruitment of perivascular mural cells. The vessel wall is therefore of poor structural integrity and as a result the vessels are highly leaky and prone to disintegration leading to edema, hemorrhaging and high interstitial fluid pressure (Fig. 1.5). Such conditions further accelerate pro-angiogenic inflammation and inhibit effective perfusion leading to hypoxia [114]. Forcing vascular maturation, while leading to a stabilized vasculature that is difficult to get rid of, and which support perfusion and thereby oxygen and nutrient delivery, which may not intuitively be a desired outcome in for example cancer treatment, is, however, emerging as an effective alternative strategy as compared to the aim of completely obliterating all vessels in the tumor. Especially when combined with cytotoxic agents, or radiation therapy, both of which require efficient perfusion for maximal effects, such vascular maturation therapy might act synergistically to inhibit tumor growth rate (or even induce tumor regression) and importantly make the disease manageable compared to high-dose anti-angiogenic therapy which might lead to reduced effect of other treatment and could potentially even accelerate progression to metastatic disease, a risk that is intimately association with tumor hypoxia.


Fig. 1.5
Pathological phenotypes of blood vessels. In healthy tissues most capillaries (shown in red) are covered with pericytes (shown in green; top-left image), are relatively non-leaky, quiescent and exhibit uni-directional blood flow (lower-left image). In pathological conditions, however, pericyte coverage is diminished, vessels start leaking plasma into the tissue and non-directional hyper-sprouting as a part of ongoing angiogenesis is apparent (top row). Perfusion is also impaired as vessels may support flow in both directions (lack of arterial-venous identity), are often collapsed and non-perfused, or develop futile loops which do not support efficient perfusion through the tissue (lower row)
Lymphangiogenesis and Lymphatic Specification
Arteries and capillaries in healthy tissues are generally slightly leaky, which is the main mechanism of interstitial fluid production. Interstitial fluid, proteins, cellular and other types of debris is in turn collected by lymphatic vessels, a special vasculature that functions as the “sewage system” of the organism. The movement of fluid from arteries and capillaries to lymphatics is, however, also important for efficient delivery of nutrients and other factors through the tissues. The lymphatic vessels move the collected liquid and materials through an entirely afferent system, the content of which is eventually emptied into the cardinal vein at the duct of Cuvier, thereby transporting the liquid back into the blood from where it came. As such, the lymphatic vessels are essential for liquid homeostasis in the tissue and inefficient lymphatic functions or defective lymphatic developmental syndromes are primarily characterized by an accumulation of fluids (i.e. edema) [118]. As the interstitial fluid is rich in foreign proteins at sites of infection, the lymph (liquid in the lymphatic vessels) is first moved through the lymph nodes, where it bathes resident antigen-presenting dendritic cells, which in turn activate T- and B-cell responses against the invading pathogens. As such, lymph nodes both contribute to interstitial fluid drainage as well as adaptive immunity through being an integral component of the lymphatic system.
The lymphatic vessels are structurally and histologically different from blood vessels. Lymphatic capillaries emerge in blind-ended sacs in which the interstitial fluids are collected. As flow can only occur in one direction, the liquids will constantly be removed from the sacs, leading to a negative pressure which enables efficient “suction” and thus drainage of fluids [119]. The lymphatic endothelial cells in the capillary region has discontinuous and patchy basement membrane, which is not in direct contact with the lymphatic endothelial cells but rather is connected via filaments rendering the lymphatic endothelium naked. Furthermore, the lymphatic endothelial cells have no tight junctions but overlap one another in structures that function as valves only allowing liquid and macromolecules, cellular components or whole cells to flow in, but not out of the vessels. Just as in large veins, there are also valves in the lumens of the lymphatic vessels, which secure directional flow important for maintaining the negative pressure in the lymphatic sacs. Lymphatic capillaries drain into larger collecting lymphatic vessels, which are covered with smooth muscle cells that aid in the transport of lymph towards the lymph nodes and later the ducts of Cuvier [120]. The special structure of the lymphatic vessels is mirrored by a specific profile of genes being expressed in these vessels, which provide means to distinguish lymphatic vessels from blood vessels. The VEGF receptor three is a frequently used lymphatic-specific marker [26], although it has recently been found expressed also by tip cells in actively expanding blood vessels. However, as the lymphatic vessels are morphologically very different from tip cells, it is still convenient to use VEGFR3 staining as a histological tool. Lymphatic but not blood vessel endothelial cells also express the membrane glycoprotein LYVE-1, the transcription factor responsible for lymphatic endothelial identify, Prox1 and the adhesion protein Podoplanin [121, 122].
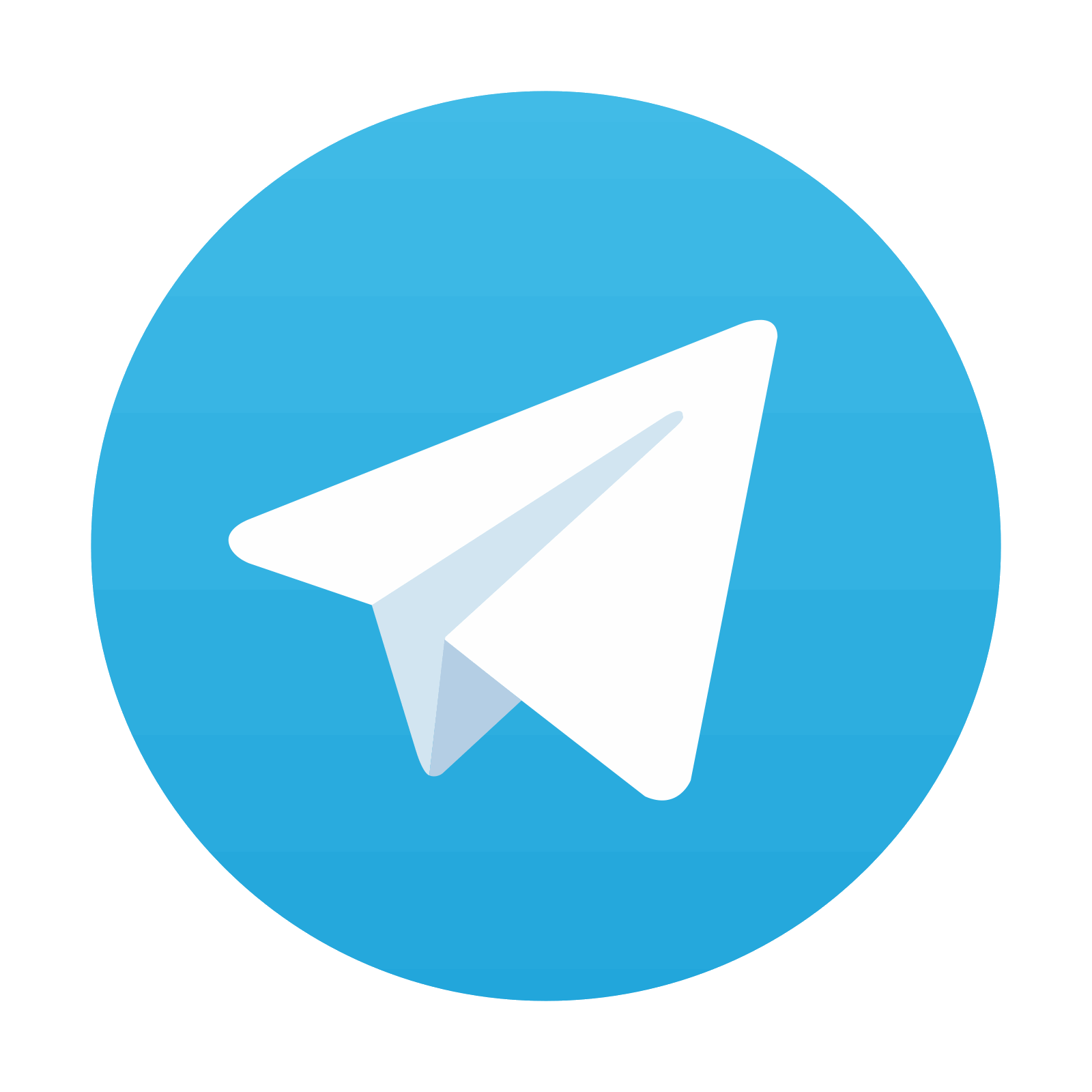
Stay updated, free articles. Join our Telegram channel
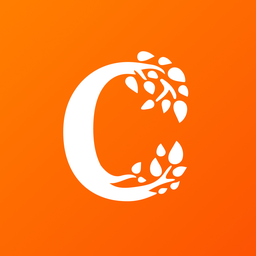
Full access? Get Clinical Tree
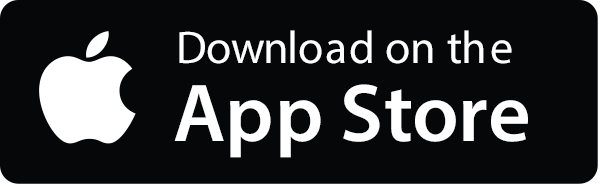
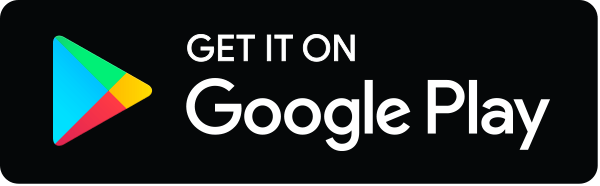