Fig. 8.1
The dermal texture of human skin changes substantially with age. Young (buttock of a 27-year-old male) (a, c, e, g) versus photoaged skin (cheek of a 75-year-old female) (b, d, f, h) processed for different histological and immunofluorescence stainings, respectively: (a, b) hematoxylin and eosin staining for morphological survey; (c, d) Movat-pentachrome staining for the simultaneous demonstration of different tissue components (cell nuclei in dark blue, cytoplasm in red, collagens in yellow, GAGs in light blue); (e, f) resorcin fuchsin with thiazine-picric acid staining for the visualization of elastic fibers in black-violet, collagen fibers in red, and nuclei in dark brown; and (g, h) immunofluorescence analyses for the localization of elastin in red and fibrillin in green with blue counterstain for cell nuclei. The intense indentation of the DEJ zone of young skin has largely disappeared in old skin (a, b). Collagen fibers are homogenously distributed and enriched in the dermal str. reticulare of young skin but diminished in the photoaged skin where few condensed fiber bundles persist (c, e vs. d, f). Oppositely, GAGs are enhanced in the dermis of photoaged in contrast to young skin (c, d) as is the staining for elastin, which, however, appears aggregated in the photoaged dermis (e, f). The organization of elastin fibers has completely changed towards amorphous aggregates with increased elastin content in photoaged versus young skin (g, h). The loss of elastin fibers and the accumulation of elastotic material (red) is particularly obvious in (h), whereas fibrillin-containing microfibrillar assembly (green) seems, at least in part, to be maintained. Bars in (f) (for a–f) and in (h) (for g–h) = 100 μm
In sun-damaged skin, also melanocytes are adversely affected. They are irregularly arranged throughout the basal epidermal layer and show strong variation in size, morphology, and melanin content. This explains the appearance of hyperpigmentation despite the fact that the number of melanocytes declines (Breathnach and Wyllie 1964; Gilchrest et al. 1979).
The most prominent changes in extrinsically aged skin are, however, seen in the dermis where the severity of changes correlates with the degree of UV damage. Solar elastosis is the most obvious and well-described alteration of the ECM (Kligman 1969). It describes an accumulation of fragmented elastic fibers (Mitchell 1967). Although the exact composition is not yet known, elastin and fibrillin, the main components of the elastic fibers, as well as fibronectin and GAGs could be detected histochemically (Chen et al. 1986; Montagna et al. 1989; Mera et al. 1987; Sams and Smith 1961), as also illustrated in Fig. 8.1. It is suggested that the fragmentation of GAGs in areas of solar elastosis contributes to loss of their hydrating properties (Bernstein et al. 1996b; Waller and Maibach 2006; Tzellos et al. 2009). In photoaged dermis, the content of collagen types I and III is generally decreased (Griffiths et al. 1993; Warren et al. 1991; Bernstein et al. 1996a), and it is discussed that it is replaced by the elastotic material (Fig. 8.1c–f). Accordingly, mature collagen fibers can no longer be detected (Bernstein et al. 1996a; El-Domyati et al. 2002; Schwartz et al. 1993) or are very thin and partly fragmented (Scharffetter-Kochanek et al. 2000). Different from intrinsically aged skin, the number of fibroblasts increases, and they adopt a stellate phenotype with a highly activated rough endoplasmic reticulum, indicative for increased metabolic activity (Uitto 1986). In addition, mast cells and mononuclear cells are attracted in extrinsically aged skin (Lavker and Kligman 1988; Krutmann 2003; Gunin et al. 2011b).
8.4 Mechanism of Aging
What are the molecular mechanisms underlying the aging process of the skin? A detailed review on mechanisms causing fibroblast aging was recently provided (Tigges et al. 2014). As we hypothesize that the major target of skin aging is the postmitotic tissue of the dermis rather than the constantly renewing epidermis, we here largely concentrate on mechanisms contributed to dermal aging.
8.4.1 Signaling Cascades and MMPs
Many studies showed that intrinsic and extrinsic skin aging have some common features, like the engagement of the same signaling pathways (Fisher et al. 2002). For example, in both cases, the MAPK pathway is dysregulated and so are the MAP kinases JNK and p38 age-dependently overexpressed (Chung et al. 2000; Fisher et al. 1998). The activation of these kinases upregulates the transcription factor AP-1 (Sardy 2009; Fisher et al. 2002), leading to an increased expression of the matrix-metalloproteinases MMP-1, MMP-3, and MMP-9, which are instrumental in the degradation of a lot of ECM components and matrix remodeling (Fisher et al. 1996, 2002; Angel et al. 2001). Furthermore, the effect of matrix degradation can be intensified by the potential of AP-1 to also downregulate the synthesis of dermal collagen by blocking the effects of TGFβ (Fisher et al. 2002).
8.4.2 Theory of Free Radicals/ROS
Free reactive oxygen species (ROS) are endogenously formed or exogenously induced and play a major role in extrinsic and intrinsic skin aging. It was shown that the ROS level in skin rises with age, while the cellular mechanisms to reduce the ROS level decline (Makrantonaki and Zouboulis 2007; Rhie et al. 2001).
High ROS level leads to the activation of the MAPK signaling pathway by activating some surface receptors like for EGF, TNF-α, insulin, and IL-1 and in turn causing upregulation of the MMPs, as it was described before (Klotz et al. 1999). Thus, a high ROS level could trigger MAPK signaling, AP-1 activation, and MMP expression thus being causal for the high amount of degraded collagen in aged skin. The main source for ROS in intrinsically aged skin is the mitochondria. About 1–5 % of the oxygen input is converted into ROS under normal physiological conditions, and it was shown that with age defects in the mitochondrial respiratory chain lead to a further increase of the ROS level (Trounce et al. 1989; Yen et al. 1989). In UV-exposed skin, additional ROS is produced by energy transfer from UV-absorbing chromophores, such as NADH−/NADPH, tryptophan, riboflavin, or trans-urocanic acid (Hanson and Simon 1998), thereby explaining the even higher amounts of MMPs and consequently the increase in matrix degradation.
One important aspect in this context is the mutation rate of mitochondrial DNA (mtDNA). Due to its unprotected state (histone-free), mitochondrial DNA is especially susceptible to oxidative damage. As the mtDNA mainly codes for proteins being part of the oxidative phosphorylation, mutations of the mtDNA lead to defects and further increase the production of ROS; a vicious circle is established (Camougrand and Rigoulet 2001; Ozawa 1995).
It is suggested that mtDNA mutations play a role in intrinsic as well as in extrinsic skin aging. However, in extrinsically aged skin, clearly higher levels of mtDNA mutations are achieved (Berneburg et al. 1997). The causal relation between mt mutations and extrinsic aging could be confirmed by in vitro studies using fibroblasts bearing constitutive mtDNA mutations that impaired the function of the respiratory chain. Impressively, those cells, when cultured in skin equivalents, mimicked the abovementioned features of extrinsic aging, including MMP overexpression, and reduced support of keratinocyte proliferation (Krutmann 2011; Majora et al. 2009).
8.4.3 Cellular Aging, Replicative Senescence, and the Role of Telomeres
Even under optimal conditions, fibroblasts in cell culture have a life span limited to 50–70 cell divisions having resulted in the concept of “replicative senescence” (Hayflick and Moorhead 1961; Hayflick 1965). One potential mechanism for this “Hayflick limit” came about with the description of the end replication problem (Olovnikov 1973; Watson 1972) that during every round of replication leads to loss of 50–200 nucleotides from the 5’-ends of linear chromosomes, the telomeres (Harley et al. 1990; Levy et al. 1992). Nevertheless, these telomeres provide only a temporary protection as, when they progressively shorten under a critical length, the cells suffer from induction of apoptosis or senescence (Lombard et al. 2005; d’Adda di Fagagna et al. 2003; Herbig et al. 2004). Thus, critical telomere shortening is supposed to be responsible for the accumulation of senescent and functionally impaired fibroblasts in aging skin (Campisi 1996). However, this hypothesis is merely based on results with cultivated cells in vitro, and it is still controversially discussed if it is also valid for the situation in the skin in situ (Cristofalo et al. 2004; Rubin 2002; Maier and Westendorp 2009). Senescence, as commonly assessed by the expression of a specific β-galactosidase, the senescence-associated β-gal pH6.0, was originally described for aged skin (Dimri et al. 1995). However, verification still needs to be awaited. Similarly, the role of telomere loss is a matter of debate. Investigating telomere lengths in different-aged skin, an age-dependent reduction was described; however, the degree of telomere length reduction was generally very limited (Sugimoto et al. 2006; Lindsey et al. 1991). This may be attributed to the fact that the constantly regenerating epidermis expresses telomerase, the enzyme able to counteract telomere loss, and that fibroblasts only proliferate rarely in vivo, thereby bypassing proliferation-dependent telomere loss. This does not exclude that upon stress, e.g., UV radiation, telomeres of individual cells get damaged and may suffer from accelerated telomere loss (Leufke et al. 2013). However, it strongly suggests that telomere loss is not the driving force in the process of skin aging but that it is rather a consequence of damage and insufficient telomerase activity, respectively. One major factor in this damage-dependent scenario is ROS (Ayouaz et al. 2008), thus further strengthening its pivotal role in skin aging.
8.4.4 Molecular Mechanisms of Matrix Alterations
Aging of skin is accompanied by a large variety of structural changes. Because of their high half time, many matrix components are predisposed for accumulating damages and modifications leading to molecular aging. So, the half-life of fibrillar collagen is 15–95 years and for proteoglycans 11–23 years, and elastin and fibrillin persist in the tissue from embryogenesis on for the whole life of the organisms (Naylor et al. 2011).
In aged skin, proteolytic enzymes, such as MMP-1, MMP-2, MMP-3, and MMP-9, cause fragmentation of the collagens, proteoglycans, and elastic fibers (Ashworth et al. 1999; Birkedal-Hansen et al. 1993; Berton et al. 2000; Murphy and Docherty 1992). Additionally, glycation of the matrix components causes the formation of intermolecular cross-links which makes the molecules less soluble and, even more important, blocks further degradation of the fragments (Rock and Fischer 2011; Bailey et al. 1998). Finally, an increase in protein oxidation is seen, especially in UV-exposed skin where protein oxidation is induced by high ROS levels. This as well provokes the formation of protein aggregates, which resist more strongly to degradation (Sander et al. 2002). Therefore, accumulating alterations in the matrix disturb the mechanical and structural integrity of the skin and thus are most likely the molecular basis for the deteriorated appearance of aged skin.
8.4.5 Development of Solar Elastosis in Extrinsically Aged Skin
Solar elastosis, as described above, is most likely not restricted to destructive processes, i.e., degradation of preexisting elastic fibers, but it includes as well a dysregulated neosynthesis of elastin and fibrillin without proper macromolecular fiber assembly thus contributing to the accumulation of elastotic material (Jenkins 2002). The expression of the mainly responsible proteolytic enzymes like elastase or MMPs has been found to be enhanced by NF-κB, which gets activated by UV (Kohl et al. 2011). An additional consequence of dermis-specific NF-κB activation is an enhanced release of cytokines by the epidermal keratinocytes. Among these cytokines are IL1-α and GM-CSF that induce the expression of elastase in the fibroblasts (Nakajima et al. 2012; Imokawa 2008) and pro-inflammatory cytokines like IL-6, IL-8, VEGF, and TNF-α which are capable of attracting inflammatory cells (Yaar and Gilchrest 2007; Yamamoto and Gaynor 2001). These are not only per se producers of proteolytic enzymes, but also they produce and deliver ROS into their surroundings thereby extending the damaging effect on the dermal matrix. Therefore, the UV-provoked inflammatory reaction is legitimately suspected to play an important role in matrix degradation associated with aged skin (Rijken and Bruijnzeel-Koomen 2011; Rijken and Bruijnzeel 2009).
8.4.6 Loss of Mechanical Stimulation
Physiology and synthetic potential of the fibroblasts in the dermis are dependent on the tensile stress the cells are subjected to (Ingber et al. 2014). The cells sense these mechanical cues via their integrin receptors by which they are attached to the surrounding ECM, e.g., to the collagen fibrils. When they come under tensile forces, either external ones or generated by cellular contractibility, the cells adopt a stretched shape. Modification and degradation of the ECM components cause loss of adhesion points for the cells’ integrins, cellular detachment, and deprivation of the positive mechanical input that, under normal conditions, ensures the synthetic phenotype of fibroblasts (Kessler et al. 2001). In consequence, a vicious circle is started that leads to increased ROS, proteolysis and a reduction of collagen, as well as ECM neosynthesis (Fisher et al. 2008, 2009). As this process is self-energizing, the final result is a dramatic loss of ECM content and structural integrity of the dermis.
8.4.7 Estrogen Depletion
Intrinsic skin aging is fundamentally influenced by hormonal changes. In particular in females in postmenopausal age, the blood concentrations of estrogen and progesterone drop considerably, and this is accompanied by the typical features of skin aging, i.e., dryness, epidermal atrophy, diminution of collagen, loss of elasticity, wrinkle formation, and impaired wound healing (Bolognia et al. 1989; Brincat 2000; Rittie et al. 2008; Sumino et al. 2004). Most importantly, hormone replacement therapies could remarkably alleviate these problems (Rittie et al. 2008; Zouboulis and Makrantonaki 2011). Although this knowledge is still mainly empirical, several indications of underlying molecular mechanisms exist, e.g., of a specific activation of the MAPK- and insulin-signaling pathways as well as of an upregulation of protective antioxidative enzymes by the ligand-stimulated estrogen receptor (Yan et al. 2011; Jackson et al. 2011). Estrogen stimulation of old fibroblasts, on the other hand, did not abrogate expression of genes correlated with extrinsic skin aging (S. Gundermann and P. Boukamp, unpublished results), suggesting that estrogen deficiency in all its complexity stands for a major pathway in the regulation of intrinsic skin aging.
8.5 Stem Cells in Skin and Aging
Can all this be related to stem cell aging? As described, skin represents a highly complex tissue and accordingly contains a broad stem cell repertoire, including resident epidermal and mesenchymal stem cells (MSCs), migratory melanocytic stem cells derived from the embryonic neural crest (NC), and dendritic precursor cells differentiating into Langerhans cells.
8.5.1 Evidence for Epidermal Stem Cell Aging
One hypothesis of skin aging is that stem cells of the epidermis decrease in number and change their function with age, leading to the characteristic phenotype commonly described for aging skin (Weinberg 2006). However, and still largely underappreciated, most of the presently available data point to a different direction. Comparison of epidermal stem cells from young and old mouse skin neither identified significant differences in stem cell number and gene expression profile nor in telomere length (Giangreco et al. 2008; Stern and Bickenbach 2007). Bickenbach and coworkers further investigated old mouse epidermal stem cells for their ability to contribute to different tissues during development. Using a blastocyst implantation assay, they unequivocally demonstrated that neither the proliferative potential nor multipotency of the epidermal stem cells did decrease with aging (Liang et al. 2004).
Alternatively, it was recently reported that the frequency and cell cycle kinetics of the transit-amplifying (TA) cells, the proliferative active descendants of the stem cells, were altered in aged mouse epidermis. Performing long-term repopulation in vivo and colony formation in vitro, Charruyer et al. reported that TA cell frequency was increased with aging and that the aged TA cells persisted longer. This argues for age-dependent alterations in TA cell kinetics with an increase in the proportion of cycling keratinocytes, as well as an increase in cell cycle duration (Charruyer et al. 2009). Due to the experimental setup, however, it has to remain elusive whether this change in cell kinetics is intrinsically regulated in the aging TA population or whether environmental influences may be the reason for this phenotypic shift.
Using well-established murine HF stem cell models, Doles and Keyes (2013) came to a quite different conclusion. Keratin 15 (K15)-positive cells are one of the best characterized stem cell populations in the HF. Utilizing a K15 promoter-reporter mouse model, they showed that the K15 stem cell population increased with age. However, their clonogenic capacity was strongly diminished. They also showed functional impairment when measuring the response to ionizing radiation and demonstrated rapid development of skin lesions upon phorbol ester treatment. Global analysis of transcript expression demonstrated that in addition to the stem-like signature, the old stem cells were markedly altered in critical signal transduction cascades. In particular, Jak-Stat signaling was shown to be responsible for inhibiting stem cell function. In their study, Doles and Keyes for the first time provided evidence that HF stem cells are susceptible to age-associated changes and may contribute to aging phenotypes (Doles and Keyes 2013).
Unfortunately, the markers identified for murine HF stem cells do not account for human HF populations. Even more so, the identity of the stem cells of the interfollicular epidermis (IFE), which predominates in human skin, is largely elusive as recently reviewed (Boehnke et al. 2012). On the other hand, there is functional evidence that also human IFE contains a stem cell population which consists of slowly cycling cells and which comprises less than 1 % of the basal keratinocytes (Muffler et al. 2008). When activated, these cells express telomerase activity, which allow them to counteract proliferation-dependent telomere loss. Accordingly, age-dependent telomere loss appears marginal (Harle-Bachor and Boukamp 1996; Krunic et al. 2009), and therefore, it is unlikely to be a driving force of epidermal stem cell aging.
Based on early studies which demonstrated different colony-forming ability for stem cells, TA cells, and differentiating keratinocytes, it was suggested that aged human keratinocyte stem cells exhibit decreased colony-forming ability (Barrandon and Green 1987). A first study on human HF stem cells also reported on a decrease in stem cell-related holoclones with age (Lecardonnel et al. 2013). Due to the small sample size, the authors were not able to sort for potential stem cell populations and instead investigated rather undefined cell populations. Although not studied extensively, our studies do not confirm this notion. We have, as yet, no indication that keratinocytes from older donors exhibit a different growth profile as determined by their proliferation potential in 2D cultures nor that they display reduced engraftment and long-term regeneration in 3D organotypic cultures (Boukamp and Stark, unpublished). It is noteworthy that also skin grafts from older individuals can essentially outlive their donors (Gallico et al. 1984; Pellegrini et al. 1999). Thus, maintaining an appropriate functional physiology into old age seems essential for survival of epidermal stem cells. This further supports the notion that they may be different from stem cells from other tissues (Winter and Bickenbach 2009).
Taken together, the concept of inhibiting epidermal stem cell function and/or number, as a major driving force of skin aging is intriguing. However, studies addressing this issue are still limited and the findings are controversial. In consequence, further detailed studies are needed to thoroughly investigate the aging processes of mouse but particularly also of human skin.
8.5.2 Aging of the Epidermal Melanocyte and Langerhans Cells
Two other types of cells resident in the epidermis are essential protagonists in skin physiology. These are the Langerhans cells (LCs) and the melanocytes.
LCs represent the dendritic cell subset which provides the first immune barrier for invading pathogens and in addition have been implicated in tolerance induction (for review see (McKenzie et al. 2006)). They originate from bone marrow precursors and were described as a nonlymphoid tissue contingent of dendritic cells in the skin (Schuler and Steinman 1985). LCs are unique in their development compared to other dendritic cells in that they are long-lived and exhibit self-renewal capacity in the epidermis. For this reason, it is presently still unclear whether self-renewal is a trait of all LCs or whether a population of LC precursor cells exists in the epidermis that are responsible for the observed stem cell characteristic (Lako et al. 2002; Ghigo et al. 2013). It is further suggested that a local pool of proliferating hematopoietic precursor cells exist that populate the skin during embryonic development (Hoetzenecker et al. 2005; Gago et al. 2009; Schuster et al. 2009) or, respectively, that fetal liver-derived LC precursors with myelo-monocytic phenotype, similar to primitive yolk sac macrophages, contribute substantially (Hoeffel et al. 2012). Furthermore, recently two types of bone marrow-derived LCs were described, long-term and short-term LCs. Long-term LCs are transcriptionally regulated by the inhibitor of DNA binding, ID2, a TGFβ1 target gene, thus suggesting that TGFβ signaling is not only required for the development of LCs (Hacker et al. 2003) but also absolutely essential for LC homeostasis. Accordingly, TGFβ-deficient mice lack LCs completely (Borkowski et al. 1996), and TGFβ receptor-deficient mice show reduced numbers of them (Slominski and Paus 1993; Kel et al. 2010). An excellent review on the regulatory networks controlling LCs in skin was recently provided by (Hieronymus et al. 2014). Addressing LC development and function during aging of mice, no differences in the life span of LCs and apoptosis rate in aged versus young mice were found; nevertheless, their frequency and maturation were reduced in a stepwise fashion starting at 12 months of age (Xu et al. 2012). In agreement with these observations, Sprecher et al. proposed that the age-dependent decrease in LC density could result from a deficiency in local progenitors (Sprecher et al. 1990). Xu et al. also reported on a distinct aging-specific miRNA gene expression profile which might interfere with LC-related signaling pathways and elicit age-related developmental and functional defects in the LCs. In general, aging of LCs can be considered a combination of both, loss of LC number and function (Xu et al. 2012).
Similar as LCs, also melanocytes represent an important cellular fraction of the epidermis. They are specialized neural crest-derived cells that synthesize and distribute the pigment melanin packed in melanosomes to their neighboring keratinocytes. Located in the basal layer of the IFE, they spread their dendrites and contact up to 38 basal keratinocytes, thereby achieving transfer of melanosomes over a defined distance. In humans, melanocytes are located throughout all regions of the skin, i.e., the IFE, dermis, and HF, while in mouse skin, melanocytes are largely restricted to HFs with the exception of hairless regions such as ear, tail, and ventral paws. In mice, melanocyte precursor cells, the melanoblasts, are primarily formed in the neural crest during embryogenesis and then migrate through the dermis and epidermis into the bulge region of the developing HF. This process is dependent on c-kit and its ligand, the stem cell factor SCF (reviewed in (Sarin and Artandi 2007). During hair cycle, the quiescent bulge melanocyte stem cells proliferate and move into the outer root sheath of the HF (Nishimura et al. 2005). Upon differentiation and movement into the lowest part of the HF, the bulb, they gain the ability for production of melanin, which then is transferred as melanosomes into adjacent keratinocytes, thereby giving rise to the pigmentation of the growing hair.
Hair graying is a prominent feature of aging, but also in the IFE pigmentation is generally decreasing with age, as excellently reviewed by Sarin and Artandi (2007). This process is associated with loss of melanocytes and melanocyte stem cells (Steingrimsson et al. 2005). Actually, it was shown that the number of unpigmented melanocytes decreases with age rendering elderly skin (age >70 years) almost free of them (Commo et al. 2004). This implies that hair graying is due to age-dependent depletion rather than dysfunction of the melanocytes. The molecular mechanism for melanocyte depletion is still elusive. It is assumed that oxidative damage as a result of melanin synthesis may be involved, an argument supported by the fact that no melanocyte loss was seen in albino mice (Johnson and Jackson 1992). Another potential mechanism is age-dependent telomere loss, which may, upon a critical length, drive the cells into senescence or apoptosis. However, when we surveyed human skin from different-age donors for melanocytes, we did not detect that they declined from the IFE with age (Krunic et al. 2009). This questions the hypothesis that loss of melanocytes is a general consequence of skin aging. It is also of interest to note that hair graying can already occur at early age in healthy humans and may long precede the aging process of other tissues thus making these individuals an excellent cohort for studying the mechanisms behind melanocyte aging.
8.5.3 Stem Cell Aging in the Dermis
Last but not least, dermal stem cells are supposed to contribute to skin aging. Unfortunately, stem cells in the dermis have been characterized poorly, as yet, although the dermis represents a larger reservoir for adult stem cells than epidermis and hair follicles together (Shi et al. 2006).
A minor subpopulation of stem cells in the dermis fulfills the common criteria of mesenchymal stem cells (MSCs). In general, these MSCs are considered to be a cell pool with high regenerative capacities to ensure maintenance of tissue function and homeostasis throughout the life span.
In 2001, Toma et al. were the first to isolate MSCs from the murine dermis, and they called them skin-derived precursor cells (Toma et al. 2001). Some years later, multipotent stem cells were also isolated from the human dermis (Chen et al. 2007; Lorenz et al. 2008; Lysy et al. 2007; Toma et al. 2005). Besides differentiating into cells of the mesenchymal lineage, cutaneous MSCs are also able to differentiate in vitro into insulin-producing pancreatic cells (Shi and Cheng 2004), hepatocytes (Lysy et al. 2007), and keratinocytes (Crigler et al. 2007; Medina et al. 2006). Furthermore, MSCs were shown to trans-differentiate in vitro into neurons, Schwann cells, and astrocytes (Toma et al. 2001, 2005; Dyce et al. 2004; Gingras et al. 2007; Shih et al. 2005; Fernandes et al. 2006; McKenzie et al. 2006).
In skin, MSCs have been localized in the connective tissue sheath surrounding the HF, the follicular papilla, and in the adipose tissue of the hypodermis (Jahoda et al. 2003; Lako et al. 2002; Hoogduijn et al. 2006).
The mechanisms of MSC aging in general are described in detail in Chap. 11, so that we here only refer to dermally derived MSCs. Accordingly, Gago et al. reported on an age-dependent functional depletion of the stem cell pool in the human skin (Gago et al. 2009). In their study, they analyzed skin-derived precursors isolated from more than 100 individuals from 8 months to 85 years and finally showed that the aging process diminished their cell pool and/or their differentiation potential (Gago et al. 2009). Although the underlying mechanism is unclear, this age-dependent decline could contribute to an impaired tissue homeostasis.
The “classical” bone marrow-derived MSCs are defined by their potential to differentiate into three linages, i.e., bone, cartilage, and fat (da Silva Meirelles et al. 2008; Chen et al. 2007). Unfortunately, molecular markers differentiating dermal MSCs from fibroblasts are rare. A recent study comparing the expression profile of MSCs from bone marrow, skin, and adipose tissue with dermal fibroblasts demonstrated that fibroblasts express the same immune-phenotypic markers and the same gene transcripts that are known to be expressed in stem cells (Brohem et al. 2013). Since selection for MSCs is presently still restricted to selective media (MSC basal medium), a certain bias remains. It is also noteworthy that also the crude mass population of dermal fibroblasts is able to differentiate into the three abovementioned lineages (Junker et al. 2010; Brohem et al. 2013). This finding strongly argues for the high intrinsic plasticity of fibroblasts and casts major doubts on our present understanding of dermal MSCs. Correspondingly, we have substantial evidence for dermal fibroblast to promote the aging process (Tigges et al. 2014). In particular, we can now show that extrinsic skin aging induces a phenotype that is best described by programming of the dermal fibroblasts to a chondrocyte trans-differentiation phenotype (Gundermann et al. 2015). As this specific phenotypic switch relies on a rise in TGFβ, the age-dependent upregulation of TGFβ is apparently an important molecular trigger in the process of extrinsic and potentially also intrinsic skin aging. Moreover, we found that fibroblasts having undergone this phenotypic switch drastically lose their potential to support epidermal keratinocytes in 3D organotypic cultures, which prompted us to hypothesize that the dermal fibroblasts are in the driver’s seat at the process of skin aging.
8.6 Conclusions
Skin aging is a multiplex process as it encompasses a multitude of endogenous changes still poorly understood as well as numerous extrinsic noxae attacking the tissue, inducing molecular damage, and triggering cellular responses, which become superimposed on the intrinsic changes. We propose that physical impacts mainly hit the dermal fibroblasts in skin that are then reprogrammed to become drivers in the aging process while the epidermal keratinocytes are merely responders being less susceptible to damage than the fibroblasts.
Whether there is a particular role for stem cell aging remains a matter of debate. There is still an urgent need of improved understanding of the different stem cell types that requires reliable means to precisely define, isolate, and maintain pure stem cell populations. Indeed, neither MSCs nor epidermal stem cells are at present sufficiently well defined for human skin (reviewed in Boehnke et al. 2012), thus making all statements about stem cell aging in human skin still highly biased. We even would go further and challenge the concept that aging must affect stem cells directly. Protection and support of the dermal fibroblasts may be sufficient to prevent many of the signs commonly related to skin aging. Clearly, this cannot provide a total prevention of aging skin. The fact that the menopausal decline in skin texture caused by estrogen depletion can be largely reverted by hormone replacement therapy (for review see (Thornton 2013)) suggests that certain aspects of the aging process can be modulated – presumably by amelioration of the cellular microenvironment. In line with this, skin aging is definitively a more severe process in females than in males, thus questioning the general approach in searching for global molecular programs in aging of the different tissues without the gender aspect being accounted precisely and carefully.
Acknowledgment
The authors are grateful to Monika Bock for her help in editing the manuscript. This work was supported by grants from the German Research Foundation (SFB873) and the BMBF GerontoSys Stromal Aging (031.5576B) and UVA Kompetenzverbund (02NUK003A).
References
Alberts B, Johnson A, Lewis J, Raff M, Roberts K, Water P (2004) Molekularbiologie der Zelle, vol 4. Wiley-VCH Verlag GmbH, Weinheim
Angel P, Szabowski A, Schorpp-Kistner M (2001) Function and regulation of AP-1 subunits in skin physiology and pathology. Oncogene 20(19):2413–2423. doi:10.1038/sj.onc.1204380 PubMed
Ashworth JL, Murphy G, Rock MJ, Sherratt MJ, Shapiro SD, Shuttleworth CA, Kielty CM (1999) Fibrillin degradation by matrix metalloproteinases: implications for connective tissue remodelling. Biochem J 340(Pt 1):171–181PubMedCentralPubMed
Ayouaz A, Raynaud C, Heride C, Revaud D, Sabatier L (2008) Telomeres: hallmarks of radiosensitivity. Biochimie 90(1):60–72. doi:10.1016/j.biochi.2007.09.011 PubMed
Bailey AJ (2001) Molecular mechanisms of ageing in connective tissues. Mech Ageing Dev 122(7):735–755PubMed
Bailey AJ, Paul RG, Knott L (1998) Mechanisms of maturation and ageing of collagen. Mech Ageing Dev 106(1–2):1–56PubMed
Barrandon Y, Green H (1987) Three clonal types of keratinocyte with different capacities for multiplication. Proc Natl Acad Sci U S A 84(8):2302–2306PubMedCentralPubMed
Baumann L (2007) Skin ageing and its treatment. J Pathol 211(2):241–251. doi:10.1002/path.2098 PubMed
Berneburg M, Gattermann N, Stege H, Grewe M, Vogelsang K, Ruzicka T, Krutmann J (1997) Chronically ultraviolet-exposed human skin shows a higher mutation frequency of mitochondrial DNA as compared to unexposed skin and the hematopoietic system. Photochem Photobiol 66(2):271–275PubMed
Berneburg M, Plettenberg H, Krutmann J (2000) Photoaging of human skin. Photodermatol Photoimmunol Photomed 16(6):239–244PubMed
Bernstein EF, Chen YQ, Kopp JB, Fisher L, Brown DB, Hahn PJ, Robey FA, Lakkakorpi J, Uitto J (1996a) Long-term sun exposure alters the collagen of the papillary dermis. Comparison of sun-protected and photoaged skin by northern analysis, immunohistochemical staining, and confocal laser scanning microscopy. J Am Acad Dermatol 34(2 Pt 1):209–218PubMed
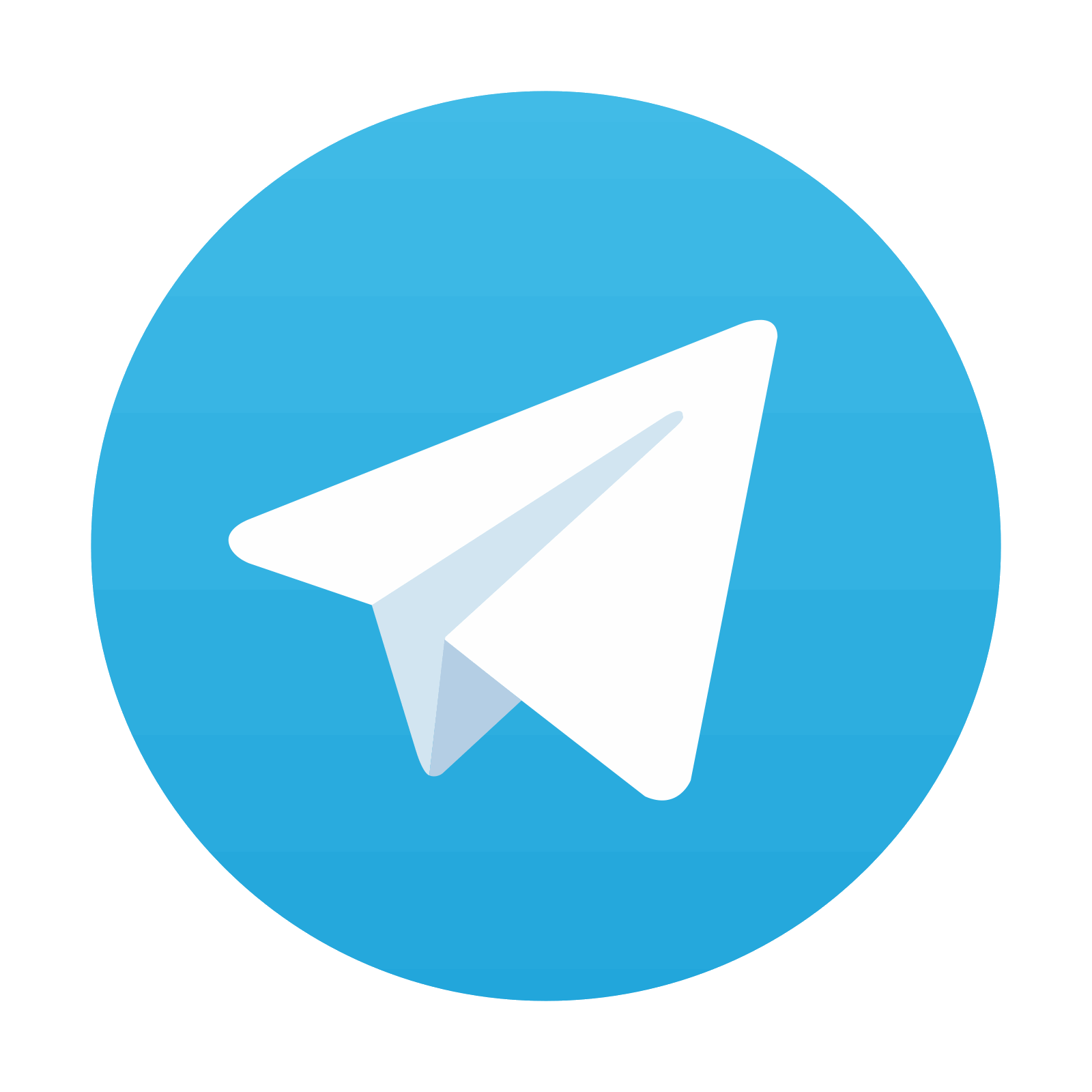
Stay updated, free articles. Join our Telegram channel
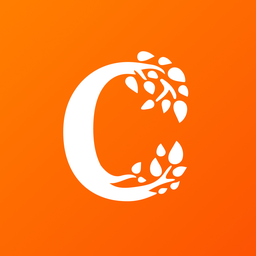
Full access? Get Clinical Tree
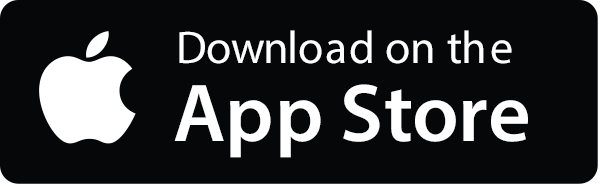
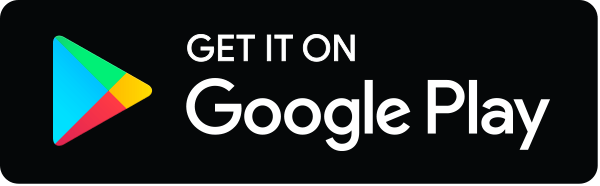