Fig. 10.1
Human muscle cross sections stained for the muscle stem cell-specific marker Pax7 (red), nuclei (blue-DAPI), basal lamina (orange-laminin), and the muscle cytoskeletal protein desmin (green). The arrows denote the anatomical location of the muscle stem cell between the myofiber membrane (sarcolemma) and the basal lamina. The first panel shows the muscle stem cell below the basal lamina, and the second panel shows that the muscle stem cells are between the myofiber and the basal lamina. The third panel verifies that the muscle stem cell is indeed between these two structures (hole where the Pax7+ cell is sitting). The last panel confirms that the nuclear marker used to identify the muscle stem cell (Pax7) is indeed co-localizing with the nucleus of a cell
After sufficient rounds of proliferation, myoblasts begin to downregulate Myf5 and upregulate MRF4 and myogenin. Both MRF4 and myogenin are involved in the specific events that orchestrate myogenic differentiation at both the transcriptional and epigenetic level (Punch et al. 2009; Rhodes and Konieczny 1989). Both MyoD and MRF4 act upstream of myogenin (Cao et al. 2006; Rhodes and Konieczny 1989; McKinnell et al. 2008), inducing the expression of myogenin and initiating terminal differentiation. The temporal expression of these MRFs was confirmed in culture demonstrating that MRF4 upregulation precedes myogenin and the upregulation of myogenin is immediately followed by terminal differentiation of myoblasts into nascent myotubes (Smith et al. 1994a). Thus upon activation, a quiescent satellite cell begins to express Myf5, followed by both Myf5 and MyoD. Following proliferation, the majority of proliferating satellite cells (myoblasts) downregulate Myf5 and upregulate MRF4 followed by the upregulation of myogenin leading to terminal differentiation and fusion of these cells either with the muscle fiber or by fusion with each other to form nascent myotubes. A subset of myoblasts may lose (or never upregulate) MyoD and withdraw from the cell cycle, repopulating the satellite cell pool through self-renewal.
Regulation of the postnatal myogenic program is thought to be controlled by a specific homeodomain protein Pax7. Pax7 is a member of the paired box (Pax) transcription factor family and has been shown to be important for normal maintenance of the satellite cell population (Seale et al. 2000). Currently, the precise role of Pax7 in myogenic specification remains somewhat unclear. Although some studies suggest that Pax7 is not essential for postnatal muscle growth and hypertrophy in the short term (McCarthy et al. 2011), recent data demonstrate the indispensable role of Pax7 in normal developmental myogenesis, postnatal myogenesis, and importantly the regenerative response to muscle injury (von Maltzahn et al. 2013; Lepper et al. 2011; Gunther et al. 2013). Pax7 associates with a specific histone methyltransferase complex that directs the methylation of histone H3K4 to induce chromatin modifications, which stimulates the transcription of Myf5, thus regulating entry into the myogenic program (McKinnell et al. 2008). Mice with an inducible knockdown of Pax7 display a progressive loss of satellite cells that is associated with severe muscle atrophy (von Maltzahn et al. 2013). The loss of cellular function in induced Pax7 null satellite cells is a result of cell cycle arrest and MRF dysregulation, demonstrating that Pax7 is an essential regulator of normal satellite cell function. Interestingly, mice with a complete genetic lack of the Pax7 gene (Pax7 −/− ) from conception have normal embryonic myogenesis mainly due to the increased activity of the Pax7 orthologue: Pax3 (Buckingham and Relaix 2007). However, Pax7 −/− mice demonstrate decreased body weight and inhibited growth and die prematurely (Seale et al. 2000). Although initially these mice were thought to lack satellite cells completely (Seale et al. 2000), it was later confirmed that they do in fact possess satellite cells, albeit at low numbers (Oustanina et al. 2004), and these cells are rapidly lost as the animals age (Relaix et al. 2005). Thus, it appears that Pax7 is instrumental in the regulation of satellite cells but may not be necessary for postnatal myogenic specification (Relaix et al. 2006). In addition Pax7 appears to be necessary for satellite cell self-renewal (Olguin and Olwin 2004). In a series of studies examining the potential role of Pax7 in satellite cell self-renewal, the forced expression of Pax7 induced cells to exit the cell cycle and return to quiescence. Furthermore, Pax7 was found to be absent in myogenin-positive cells illustrating a potential reciprocal inhibition between Pax7 and myogenin, suggesting that Pax7 must be downregulated in order for differentiation to proceed (Olguin and Olwin 2004; Olguin et al. 2007). Further work by Zammit et al. (Zammit et al. 2004) demonstrated that Pax7 is co-expressed with MyoD in proliferating myoblasts and that the downregulation of Pax7 in these cells coincided with the progression of cells into terminal differentiation, while a subset of cells downregulate MyoD but retain Pax7 and withdraw from the cell cycle, thus replenishing the satellite cell pool. Collectively, these studies suggest that Pax7 is involved in the coordination of normal postnatal myogenesis. Pax7 appears to be instrumental in the initiation of the myogenic program through the transcriptional activation of Myf5 and maintenance of the satellite cell pool by increasing cell survival and preventing differentiation, thus favoring self-renewal, at least in embryonic and early postnatal myogenesis (McKinnell et al. 2008; Gunther et al. 2013; Relaix et al. 2006; Olguin and Olwin 2004).
In adult life muscle fibers are generally stable, requiring only minor myonuclear turnover due to subtle acquired structural or nuclear damage (Decary et al. 1997). Estimates for myonuclear turnover suggest that approximately 1–2 % of myonuclei are replaced on a weekly basis in rat muscle (Schmalbruch and Lewis 2000). If we extend the observation that a rat extensor digitorum longus (EDL) has approximately 2.2 million myonuclei (Viguie et al. 1997), then the average satellite cell-mediated myonuclear turnover in the EDL is ~22,000 myonuclei per week. Therefore even in the absence of myotrauma, the role of the satellite cell appears to be indispensible. Furthermore, muscle lacking functional satellite cells due to exposure to radiation is unable to undergo significant hypertrophy in response to chronic overload (Adams et al. 2002). This study demonstrates that satellite cell-mediated myonuclear addition is necessary to support significant skeletal muscle hypertrophy. However, this theory was recently refuted by data, which illustrates that satellite cells are not required for short-term muscle hypertrophy (McCarthy et al. 2011). Muscle hypertrophy was achieved in satellite cell-depleted muscle suggesting, in the short term, that intrinsic protein synthetic mechanisms are sufficient to support significant muscle hypertrophy. Skeletal muscle is an incredibly plastic tissue with significant adaptive potential, and hypertrophy is achieved without myonuclear accretion from satellite cell fusion in the short term (2 weeks). However, in the long term there is an attenuation of hypertrophy in muscle lacking satellite cells (Fry et al. 2014), an impaired regenerative capacity, as well as an increase in tissue fibrosis or extracellular matrix expansion (Fry et al. 2014, 2015). In addition, in satellite cell ablation studies, the control groups demonstrate muscle hypertrophy associated with satellite cell activation and fusion with myofibers, which suggests that the normal physiological response to overload is a combination of increased myofiber protein synthesis and satellite cell-mediated myonuclear accretion (McCarthy et al. 2011; Wang and Rudnicki 2012). Although muscle hypertrophy can occur without satellite cells in the short term, the absence of satellite cells completely prevents muscle regeneration following muscle injury. Several recent studies using different methods of satellite cell ablation have demonstrated that, in response to toxin-mediated injury and the more physiologically mediated forced exercise injury model, skeletal muscle fails to regenerate (McCarthy et al. 2011; Lepper et al. 2011; Murphy et al. 2011; Sambasivan et al. 2011). Collectively, these studies also indicate that when the satellite cell population is ablated below a threshold level, muscle regeneration is severely impaired. This lends support to the theory that when the satellite cell pool is depleted below a certain level, the in vivo regenerative capacity of the muscle is impaired. This phenomenon is observed in diseases such as Duchenne muscular dystrophy, where the satellite cell pool becomes exhausted. Whether or not depletion of the satellite cell pool is implicated in muscle aging remains controversial. Central to our understanding of muscle stem cell function is the regulation of this cell population by extrinsic factors and is the focus of intense study in the context of normal growth and dysfunction in aging.
10.5 Regulation of Muscle Stem Cells
Mature skeletal muscle is known to produce a host of growth factors such as insulinlike growth factor-1 (IGF-1) and hepatocyte growth factor (HGF) following a physiological stimulus such as overload or myotrauma following injury. IGF-1 has been shown to activate protein synthetic machinery by its interaction with the mammalian target of rapamycin (mTOR) (Frost and Lang 2011) and is a potent mitogen believed to be involved in cell recruitment to aid in tissue repair (Mourkioti and Rosenthal 2005). The expression of the MRFs appears to be regulated, in part, by the activity of locally produced isoforms of IGF-1 (Mourkioti and Rosenthal 2005; Chakravarthy et al. 2000). IGF-1 is a growth factor found in the circulation, produced in the liver and locally by the muscle fiber itself (Mourkioti and Rosenthal 2005). Three specific isoforms of IGF-1, IGF-1Ea, IGF-1Eb, and IGF-1Ec, have been described in muscle, and each may contribute to some extent to muscle regeneration/repair (Chakravarthy et al. 2000; Rotwein et al. 1986; Yang et al. 1996). IGF-1Ea transcribes the full-length IGF-1 protein, which is identical to that produced by the liver (Yang et al. 1996). IGF-1Eb may be involved in hypertrophy or muscle regeneration; however, the physiological role of IGF-1Eb in human skeletal muscle is currently unknown. IGF-1Ec or mechano growth factor (MGF) is a splice variant of IGF-1Ea that may have both autocrine and paracrine functions, stimulating satellite cell proliferation and muscle hypertrophy following muscle stretch or muscle damage (Yang et al. 1996; Hill and Goldspink 2003).
IGF-1 has been shown to activate cultured porcine satellite cells through an mTOR-dependent mechanism (Han et al. 2008) and enhance the proliferative potential of murine satellite cells by activating the phosphatidylinositol 3′-kinase/Akt signaling pathway (Galvin et al. 2003). In response to exercise or injury, IGF-1 splice variants increase within 24 h after injury in rats (Hill and Goldspink 2003; Hill et al. 2003); however, results from human studies are conflicting.
In humans, IGF-1Ec expression following isometric knee exercise can increase in as little as 2.5 h (Greig et al. 2006), suggesting that IGF-1Ec may be important for satellite cell proliferation. Information regarding the response of IGF-1Ea to exercise is equivocal, with some animal and human work demonstrating significant increases in IGF-1Ea expression as early as 24 h post-exercise or muscle damage (Hill and Goldspink 2003; Hill et al. 2003; Greig et al. 2006; Hameed et al. 2003), while others show a marked decrease in IGF-1Ea and total IGF-1 from 4 to 12 h post-exercise that remained unchanged after 96 h (Psilander et al. 2003; Bickel et al. 2005). In humans, the IGF-1 splice variants at the mRNA level (McKay et al. 2008) and the whole muscle protein level (Philippou et al. 2009), co-localized with Pax7 (using immunofluorescent techniques) (McKay et al. 2008), appear temporally related to MRF expression. IGF-1Ec is temporally co-expressed with Myf5, while IGF-1Ea and Eb are temporally co-expressed with MRF4, suggesting that alternate splicing of IGF-1 is associated with different phases of satellite cell progression (McKay et al. 2008; Philippou et al. 2009). Therefore, based on human and animal studies, it appears that IGF-1 may be a critical regulator of the myogenic response.
Other growth factors have been shown to influence the proliferative phase or differentiation of satellite cells in animals or in cell culture. Figure 10.2. shows a schematic representation of some of the major molecular regulators of the myogenic program and illustrates the timing of the MRFs with respect to the progression of satellite cells from quiescence to differentiation. These extracellular signaling molecules include basic fibroblast growth factor (bFGF), which has been demonstrated to induce activation/proliferation and inhibit differentiation (DiMario et al. 1989); nitric oxide (NO), which has been implicated in satellite cell activation, acting upstream of HGF to mediate the release of HGF (Tatsumi et al. 2002); Delta-1, which increases proliferation (Conboy et al. 2003); and myostatin and TGF-β, which inhibit activation and differentiation and maintain quiescence (McCroskery et al. 2003; Allen and Boxhorn 1987). Recently, angiotensin II (ANGII) has been implicated as an activator of quiescent satellite cells in vivo and in culture (Johnston et al. 2010). The actions of ANGII appear to regulate satellite cells through the angiotensin II receptor sub-type 1 (AT1), and may also play an instrumental role in satellite cell migration following muscle injury (Johnston et al. 2010). Platelet-derived growth factor (PDGF) and endothelial growth factor (EGF) also appear to increase satellite cell proliferation in culture (Hawke and Garry 2001). The stark reality is that there are many factors that influence satellite cell regulation, many of which are likely yet to be discovered. This reality underlies the complexity of understanding satellite cell regulation and emphasizes the challenge of understanding age-related satellite cell dysfunction. A growing area of interest is the role that inflammation and inflammatory markers play in regulating satellite cell function. Not surprisingly, the role of inflammatory markers on satellite cell function is not well understood.
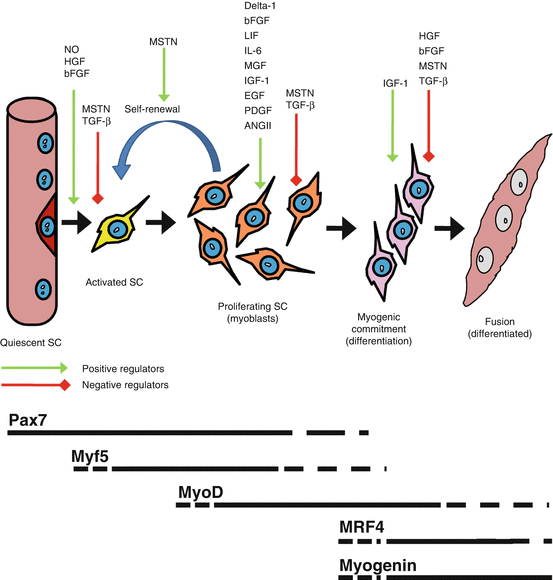
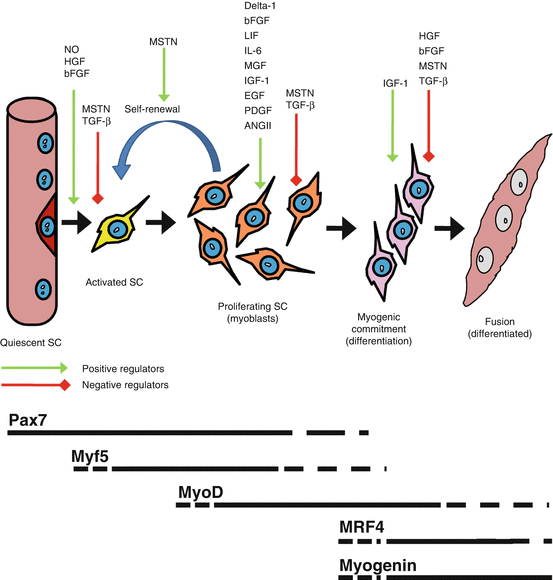
Fig. 10.2
The myogenic program from a quiescent muscle stem cell (SC) on the periphery of the myofiber (dark red cell) which then is activated (yellow cell) and undergoes rounds of proliferation (orange cell) before progressing towards terminal differentiation by either fusing together to form nascent myotubes or fusing with existing myofibers. The green arrows denote positive regulators of each step of the myogenic program, whereas red arrows denote negative regulators of each step. Below is a representation of the appearance and disappearance of Pax7 and the myogenic regulatory factors with respect to the myogenic progression of the muscle stem cells
10.6 Inflammation and Muscle Stem Cell Regulation
In young adults, myotrauma due to exercise or injury may induce damage to contractile proteins and myofibrils, or larger ultrastructural disruptions, which initiates a significant inflammatory response (Smith et al. 1994b; Beaton et al. 2002). Macrophage infiltration, which is responsible for the removal of cellular debris, is generally accompanied by lymphocytes, which coordinate the inflammatory response and importantly release factors that stimulate satellite cell proliferation (Allen et al. 1995; Cantini et al. 1995). Invading T lymphocytes can bind adhesion molecules on damaged muscle fibers and release a host of cytokines including leukemia inhibitory factor (LIF) and interleukin-6 (IL-6). In addition, damaged muscle fibers secrete several cytokines (i.e., IL-6) and growth factors (i.e., IGF-1) that may act as chemoattractants for inflammatory cells and satellite cells, augmenting the rapid repair response (Johnston et al. 2010; Pedersen and Febbraio 2008; Broholm et al. 2008; Gallucci et al. 1998; Tomiya et al. 2004). In humans, administration of nonsteroidal anti-inflammatory medication (NSAID) after damaging exercise inhibits the exercise-induced increase in satellite cells observed in controls (Mikkelsen et al. 2009; Mackey et al. 2007), illustrating that inflammation may be a key factor regulating the satellite cell response.
Cytokines released by inflammatory cells have been shown to act on satellite cells in vitro, stimulating proliferation (Cantini et al. 1995). Of the factors released, members of the interleukin-6 family of cytokines, IL-6 and LIF, appear to be instrumental in regulating satellite cell proliferation (Cantini et al. 1995; Spangenburg and Booth 2002; Austin et al. 1992). Both LIF and IL-6 bind the gp130 receptor, activating Janus-activated kinase (JAK) 2, which induces the phosphorylation of signal transducers and activators of transcription (STAT)-3 (Spangenburg and Booth 2002; Rawlings et al. 2004). Phosphorylated STAT3 (pSTAT3) forms a homodimer (or heterodimer with other STAT proteins), which translocates to the nucleus and induces the transcription of target genes such as Cyclin D1 and cMyc, which are instrumental in the initiation of the cell cycle (Rawlings et al. 2004). Importantly pSTAT3 also targets the IL-6 gene; thus, the release of IL-6 and LIF induces the production of IL-6 in target cells, creating an autocrine/paracrine loop influencing satellite cell proliferation (Rawlings et al. 2004). Until recently these findings had only been validated in cell culture models. In a landmark study by Serrano and colleagues (Serrano et al. 2008), IL-6 was shown to be an essential regulator of satellite cell function in vivo in a murine animal model. This study provided mechanistic evidence supporting the role of IL-6 as a regulator of satellite cell proliferation and that in the absence of IL-6 (IL-6 −/− mice), satellite cell proliferation and myonuclear accretion were severely blunted (Serrano et al. 2008). Furthermore, the deficiency in proliferation observed in the IL-6−/− satellite cells could be rescued by the ectopic addition of IL-6 and IL-6-mediated proliferation via the IL-6/STAT3 axis (Serrano et al. 2008). This study provides strong evidence to support the role of inflammatory cytokines in regulating muscle stem cell activity.
Aging is associated with alterations in basal levels of circulating inflammatory mediators such as IL-6 and TNFα and a decrease in circulating growth factors. The changes associated with normal tissue aging confer a negative impact on the muscle stem cell compartment, which may impact the ability of the skeletal muscle to adapt and regenerate. The remainder of this chapter focuses on several aspects of the aging process in relation to the function and control of the muscle stem cells.
10.7 Muscle Stem Cells and Aging
Aging is a complex process and is associated with many physical and metabolic alterations that lead to the progressive loss of function over time (Roubenoff 2003). Sarcopenia, which is an age-related condition that includes a progressive loss of muscle mass and strength, leads to the loss of functional capacity and an increase in morbidity (Roubenoff 1999; Beccafico et al. 2007). The progressive nature of age-related muscle wasting leads to an increased incidence of injury and loss of autonomy, resulting in a significant loss in quality of life and increased risk of all-cause mortality (Roubenoff and Hughes 2000; Melton et al. 2000). Moreover, the economic impact of sarcopenia is staggering. Common age-related injuries are often associated with falls due to a loss of strength and stability. Falls represent approximately 65 % of injuries, 85 % of injury-related hospital admissions, and 58 % of injury-related deaths in individuals over the age of 65. These statistics translate into health-care costs of approximately $1 billion dollars annually in Canada and as high as $26 billion dollars annually in the United States (Janssen et al. 2004). Similar trends have been observed in Australia, Great Britain, and Europe. Thus understanding the cellular mechanisms that lead to the onset and progression of sarcopenia is necessary to propose effective countermeasures.
The normal aging process has a profound effect on the structure and function of the skeletal muscle. Aged muscle undergoes progressive and seemingly unavoidable atrophy, with a decreased cross-sectional area accompanied by a decrease in muscle fiber number and fiber size (Grounds 1998; Koopman and van Loon 2009). Importantly, muscle function or quality appears to be reduced in aged muscle, displaying a decrease in power and maximum force production even when corrected to cross-sectional area (Brooks and Faulkner 1988). In addition, aged muscle is far more susceptible to macro and micro damage compared to young muscle, and, central to this chapter, aged muscle displays a decreased capacity for growth and regeneration (Grounds 1998; Brooks and Faulkner 1996). Satellite cells have been implicated as a significant factor contributing to the inability of the muscle to repair/remodel. An age-related impairment of the myogenic program (either impaired activation of or progression through the myogenic program) is hypothesized to be a significant contributor to satellite cell dysfunction, leading to the onset or progression of sarcopenia. In adult muscle, satellite cells comprise only a small fraction of the total myonuclei (~2 %) yet possess an incredible proliferative capacity, capable of repairing extensive muscle injury. The size of the satellite cell pool is thought to remain relatively constant throughout adult life; however, it has been suggested that in the later stages of life, the size of the satellite cell pool begins to decline, impairing the regenerative capacity of muscle. Although it is unclear at present whether there is an age-related reduction in the satellite cell pool size, it is clear that aging is accompanied by an impairment in the response of satellite cells to myotrauma in humans (Dreyer et al. 2006; McKay et al. 2013; McKay et al. 2012) and animals (Conboy et al. 2003). Several studies have attributed both intrinsic factors of the satellite cells (Jejurikar et al. 2006) as well as extrinsic factors such as age-related alterations to the satellite cell niche (Conboy et al. 2003; Carlson and Conboy 2007) as major influences on the dysfunction of the satellite cell population with aging.
10.8 Muscle Stem Cell Pool Size and Aging
There is a progressive decline in the satellite cell pool after birth from ~30–50 % of total myonuclei to a relatively stable ~2–4 % of total myonuclei in adolescences and into adulthood. Whether or not aging is associated with a reduction in the total number of satellite cells has been the focus of many studies in animals and in humans and would be the simplest explanation as to why older adults suffer significant skeletal muscle loss. Although there is significant controversy in this area, there are several key studies in animals that highlight an age-related decline in the satellite cell pool. Shefer et al. (Shefer et al. 2006) compared the satellite cell pool in four groups of mice aged 3–6 months (young), 7–13 months (adult), 19–25 months (old), and 29–33 months (senile). Their data demonstrate a 60 % decline in satellite cells in the extensor digitorum longus (EDL) muscle between young and old mice with a further 17 % reduction between the old and senile groups (Shefer et al. 2006). Similar results were observed in the soleus muscle, and it was concluded that the satellite cell pool in the young was significantly larger than that of all the three other groups. Furthermore, when grown in culture, a more robust growth rate was observed in satellite cells obtained from young muscle as compared to senile muscle. Other studies in support of these findings have also reported decreased growth rates as well as increased susceptibility for satellite cells to undergo apoptosis in vitro and in vivo compared to the young (Jejurikar et al. 2006; Shefer et al. 2006; Day et al. 2010). Single muscle fiber analysis revealed that satellite cells, expressing the marker Pax7, were markedly reduced in aged muscle and had a reduced myogenic capacity in culture (Collins et al. 2007). In fact, approximately 10 % of aged myofibers did not possess any Pax7-positive cells (satellite cells). Importantly, the aged satellite cells demonstrated a marked inability to renew the progenitor pool undoubtedly contributing to the observed reduction in satellite cell number. A rather interesting observation by Collins et al. (2007) described a subpopulation of satellite cells that appeared unaffected by the aging process. Although the majority of aged satellite cells displayed altered myogenic properties, a population of relatively rare muscle stem cells was able to produce large colonies of functional satellite cells, which retained the ability to self-renew in vitro (Collins et al. 2007). This population likely represents a very small proportion of the satellite cell pool and may be a more primitive myogenic progenitor cell, which, for reasons unknown, has been able to avoid the adverse effects of aging. It may be that these cells represent a more primitive myogenic progenitor subpopulation, which retains a similar level of potency as satellite cells from young muscle. There is significant heterogeneity in the satellite cell population (Biressi and Rando 2010), and it is possible that, much like in the bone marrow, there is a small group of stem cells that rarely divide and whose progeny is mainly responsible for amplifying in response to stimuli and self-renewing to maintain the progenitor pool. It is of note, however, that the notion that aging is associated with a loss in the size of the satellite cell pool does not go unchallenged. Several studies do not report a decline in total satellite cell number in the muscle of aged mice (Conboy et al. 2003; Dreyer et al. 2006). This may be a result of different enumeration methods or molecular markers used to quantify the satellite cell pool or species of animal used. In humans, however, it has been unequivocally shown that aged muscle (>65 years) has a reduced satellite cell pool. Recently Verdijk et al. (Verdijk et al. 2007; Verdijk et al. 2009), using the cell marker N-CAM (neural cell adhesion molecule), demonstrated that the satellite cell pool in older adults was significantly lower compared to young men, and this was mainly a consequence of the loss of satellite cells associated with type II myofibers. This was subsequently confirmed using both Pax7 immunofluorescent analysis and flow cytometry in older adults (~70 years) (McKay et al. 2012, 2013). Collectively these studies demonstrate an approximate 35 % reduction in the satellite cell pool in 70-year-olds compared to 25-year-olds, the bulk of which is made up of a marked reduction in type II-associated satellite cells (McKay et al. 2012, 2013; Verdijk et al. 2007, 2009, 2012). These studies report an approximate 30 % reduction in muscle strength, 25 % reduction of type II muscle fiber cross-sectional area (CSA), and a 20 % lower type II fiber myonuclear domain compared to young lean body mass-matched controls. Importantly these studies provide evidence that the reduced type II fiber-associated SC content in the elderly may be an important factor in the etiology of type II fiber atrophy and progression of sarcopenia (Verdijk et al. 2007). Whether the absolute number of satellite cells is an important factor for muscle aging remains a somewhat contentious issue; however, the literature clearly demonstrates that satellite cells in aged muscle become dysfunctional as a consequence of the aging process. The dysfunction can be classified as intrinsic to the stem cells themselves or extrinsic, relating to factors in the stem cell niche and circulating factors known to regulate satellite cells. It appears that the combination of these extrinsic and intrinsic factors is important to the overall function or dysfunction of the muscle stem cell population.
10.9 Muscle Stem Cells and Aging: Intrinsic Cell Failure
Muscle stem cells remain in a quiescent state on the periphery of the myofiber and become activated when sufficient cellular cues from the microenvironment initiate entry into the cell cycle. A major factor influencing the success of this process is the ability of the cell to recognize these signals and activate the appropriate cell signaling pathways. Several studies have identified key factors that appear to be altered by the aging process. Furthermore, nuclei in newly fused myoblasts must function properly to allow for adequate tissue healing and to respond appropriately to physiological stimuli for the induction of adaptation. In aged muscle it appears that all of these aspects may be impaired to some degree. Several theories have been proposed to explain how quiescent cells outside the sarcolemma could acquire age-related damage. The most prominent of these theories involves oxidative damage to DNA and cellular components from years of exposure to reactive oxygen species (ROS) (Beccafico et al. 2007; Cefalu 2011; Mecocci et al. 1999). Satellite cells and myotubes (fused differentiated myoblasts) from aged humans display an age-dependent increase in lipid peroxidation and impaired calcium handling in in vitro experiments suggesting that acquired damage throughout life impairs the basic cellular processes in these cells which may contribute to the poor adaptive response of aged muscle (Beccafico et al. 2007).
10.9.1 Susceptibility to Death
Studies examining the proliferative ability of satellite cells isolated from aged muscle and assayed in culture illustrate a basic decrease in the activation and/or proliferative capacity of old cells lending support to the theory that aged satellite cells suffer from intrinsic failure (Conboy et al. 2003; McKay et al. 2012; Jejurikar et al. 2006; Collins et al. 2007). In culture, aged satellite cells and their progeny readily undergo apoptosis (Collins et al. 2007). In the presence of proapoptotic agents such as tumor necrosis factor-alpha (TNF-α), aged satellite cells demonstrate increased levels of activated caspases and seven to eightfold more fragmented DNA as well as a 40 % reduction in the antiapoptotic protein Bcl-2 compared to young controls (Jejurikar et al. 2006). These data suggest that under stress, aged satellite cells are less capable of entering the myogenic program and fail to resist cell death, therefore impairing the response to injury. The subset of satellite cells that survive after becoming activated is able to proliferate and differentiate similarly to those of young muscle (Shefer et al. 2006; Collins et al. 2007). This suggests that if satellite cells can resist apoptosis, the intrinsic ability of some individual cells to progress through the myogenic program is retained. However, the amplification kinetics of the satellite cell pool and total yield of satellite cell progeny is significantly impaired in aged muscle (Shefer et al. 2006; Corbu et al. 2010). In addition, the ability to repopulate the reserve pool following proliferation appears to be significantly reduced in aged satellite cells (Day et al. 2010). Interestingly, in aged animals, some muscle fibers are completely devoid of satellite cells, which may indicate that some fibers have completely exhausted their satellite cell pool due to impaired self-renewal (Shefer et al. 2006; Day et al. 2010; Collins et al. 2007). The intrinsic failure of satellite cells in aged muscle may be further compounded by acquired cellular damage. Satellite cells from aged muscle have been relatively quiescent for the majority of life, which makes them susceptible to damage from genotoxic mediators such as reactive oxygen and nitrogen species from the myofiber and from the circulation (Jang et al. 2011). The accumulation of cellular damage coupled with a reduced capacity for quiescent cells to repair damage due to lower levels of DNA repair proteins and decreased response to stress (Bohr 2002) may negatively influence the ability of satellite cells to proliferate and resist apoptosis. Although the role of accumulated cellular damage in muscle stem cells has not been fully explored, it is very likely that acquired cellular damage contributes to the reduced myogenic capacity of these cells.
10.9.2 Adoption of Alternate Cell Fates
Aged satellite cells that successfully resist death and become activated in response to injury may actually activate inappropriate signaling pathways leading to inappropriate cell differentiation. In normal myogenesis there is an important balance and temporal shift from Notch signaling to Wnt signaling pathways that orchestrate proper progression down the myogenic lineage (Brack et al. 2008). Increased levels of Wnt signaling in aged animals induce satellite cells to progress away from the myogenic lineage and adopt a fibrogenic fate (Brack et al. 2007). Increased muscle fibrosis in aging is a hallmark event of the sarcopenic process and appears to be mediated, at least in part, by an increased fibrotic differentiation of satellite cells. This not only inhibits muscle repair but also decreases the quality and function of the skeletal muscle, which is particularly devastating in advanced age where there is already a loss of functional contracting tissue. To emphasize the point, myogenic-to-fibrogenic conversion of aged muscle satellite cells was substantially reduced with the injection of DKK1, a Wnt signaling inhibitor, during muscle regeneration (Brack et al. 2007). Other signaling pathways in aged muscle satellite cells involving PPARγ, and other members of the Wnt family of proteins, have also been shown to induce an adipogenic conversion of satellite cells (Taylor-Jones et al. 2002). Although it is not fully known whether the age-related adoption of alternate fates by satellite cells actually results in greater muscle adiposity or fibrosis, this observation clearly demonstrates altered intrinsic ability of the satellite cell in advanced age. The loss of cells driven down the myogenic lineage reduces the capacity of aged muscle to respond to overload and to regenerate damaged tissue; however, intrinsic dysfunction of satellite cells represents only one potential source of muscle stem cell failure. In fact, some will argue that the intrinsic dysfunction is only a result of altered extrinsic cues driving the intrinsic response. There is certainly merit in this position as satellite cells are in constant communication with cues from the muscle fiber, surrounding cells, and systemic environment. Theoretically, any alteration in the microenvironment may have an impact on intrinsic cell function.
10.10 Muscle Stem Cells and Aging: Extrinsic Factors
The satellite cell niche and the systemic milieu have been identified as factors in age-related satellite cell dysfunction. The satellite cell niche or microenvironment that supports the satellite cell helps regulate cell activity through physical contact and direct interaction with satellite cell membrane proteins (Kuang et al. 2008; Gopinath and Rando 2008). The basal lamina is one of the main niche components, which is in constant interaction with extracellular space and circulation. It is mainly composed of type IV collagen and several structural proteins, proteoglycans, and glycoproteins such as fibronectin and laminin (Woodley et al. 1983).
10.10.1 Architectural Changes to the Niche
With aging there is a progressive thickening of the basal lamina as a result of an increased deposition of collagen (Alexakis et al. 2007), which interferes with the normal extracellular matrix (ECM) functions such as the sequestration of hepatocyte growth factor (HGF) (Tatsumi and Allen 2004) and normal diffusion of key signaling molecules. Thickening and fibrosis of the satellite cell niche may impair interactions with the systemic environment and also make it considerably more difficult for the satellite cell to degrade the ECM thus hindering satellite cell migration. The niche is also susceptible to an increase in adiposity and connective tissue deposition from an age-related increase in the fibroblast pool in the interstitial spaces of the skeletal muscle (Brack et al. 2007; Kirkland et al. 2002). This may alter the secretion of soluble factors and the sensitivity of satellite cells to systemic factors. An important component of the satellite cell niche is the presence of a blood supply in the form of capillaries associated with individual muscle fibers. Aging is associated with a reduction in the capillary network surrounding myofibers and a concomitant reduction in vascular endothelial growth factor (VEGF) expression and endothelial nitric oxide synthase (eNOS) activity, which have detrimental effects to the local vasculature and satellite cell function (Croley et al. 2005; Ryan et al. 2006). Additionally, changes to the neuronal connectivity of the skeletal muscle occur with aging (Larsson and Ansved 1995). A reduction in neuromuscular connectivity by a loss of functional motor units in aging has been shown to contribute to muscle atrophy (Larsson and Ansved 1995) which, coupled with increased fibrosis, alters the satellite cell niche architecture and may impact satellite cell function. Furthermore, a reduction in neuronal input may further impair normal satellite cell function via a reduction in electrical and neurotrophic factor stimulation (Gopinath and Rando 2008). In essence age-related changes to the architecture of the niche including thickening of the basal lamina, deposition of adipocytes, and loss of capillaries and nerves can adversely impact satellite cell function.
10.10.2 Circulating Factors
Age-related changes in the microenvironment may impair satellite cell activation (Conboy et al. 2003) and even influence muscle progenitors to adopt alternate lineages (i.e., become fibrotic (Brack et al. 2007) or adipogenic (Taylor-Jones et al. 2002)) leading to the loss of regenerative capacity of cells in the aged niche (Carlson and Conboy 2007). As mentioned above enhanced Wnt signaling in aged mice induced a shift from the normal myogenic fate to an increased propensity for fibrogenesis (Brack et al. 2007). It is entirely possible that the source of Wnt proteins in aging may originate in tissues outside of muscle circulating to the satellite cell niche. It has been shown that when young muscle satellite cells are exposed to aged serum, there is an increase in fibrogenic conversion (Brack et al. 2007). In fact, increasing a single Wnt protein, Wnt3a, in young serum is sufficient for driving a myogenic-to-fibrogenic conversion, and when administered directly into regenerating muscle, the result was increased tissue fibrosis and a reduced satellite cell proliferation (Brack et al. 2007). The most elegant of the experiments demonstrating an impaired satellite cell function as a result of an aging extrinsic environment involved parabiotic pairings of young and old mice. In experiments utilizing parabiosis, young mice and old mice are stitched together via a skin flap allowing for an anastomosis to form between the animals giving rise to essentially a single shared circulation. The idea is that this model would allow for in vivo analysis of satellite cells exposed to an aging circulation. Heterochronic (young with an old) parabiotic pairings restored the regenerative capacity of satellite cells in aged mice suggesting that age-related satellite cell dysfunction is indeed extrinsic in nature (Conboy et al. 2005). Consistent with this notion, the transmembrane protein, Notch, was implicated in age-induced satellite cell dysfunction, as it remained inactive following freeze-crush myotrauma, therefore preventing satellite cell activation (as illustrated by 75 % reduction in replicating satellite cells) (Conboy et al. 2003). The forced activation of Notch or the exposure to a young circulatory environment via heterochronic parabiotic pairing (or exposure to young serum in culture) restored the regenerative and proliferative response in aged muscle to the same levels observed to that in young animals (Conboy et al. 2003; Conboy et al. 2005). Collectively these experiments implicated Notch in age-related failure of satellite cells to activate in response to a traumatic cue. In humans, Notch signaling gene expression was impaired in both isolated satellite cell cultures and in whole muscle homogenates in muscle biopsies taken from elderly men (Carey et al. 2007). Interestingly, resistance exercise training attenuated the age-related impairment of Notch-related gene expression, suggesting that extrinsic factors can be manipulated through exercise (Carey et al. 2007). It is important to note that when human primary myoblasts were cultured with serum from either young or elderly humans, proliferation and differentiation efficiencies were not altered (George et al. 2010). Importantly, however, these experiments were carried out in young cells only, and it remains unknown whether a young circulatory environment can have a positive impact on aged satellite cells in humans.
As mentioned above altered signaling between the muscle fiber and the satellite cell, such as seen with Notch-Delta signaling, is important in dictating satellite cell function. Similarly, muscle-derived growth factors are important in normal satellite cell regulation. In the vast majority of the time, satellite cells exist in a state of quiescence maintained by the interaction of the satellite cell, muscle fiber, and other niche elements (Bischoff 1990a, b). In studies from aging mice, there appears to be an increase in muscle fiber-derived fibroblast growth factor-2 (Fgf2), which causes a subset of satellite cells to leave the quiescent state and lose the ability to self-renew (Chakkalakal et al. 2012). From this data it appears that inappropriate changes in fiber-released growth factors may be instrumental in the reduction of the satellite cell pool through inducing an inability to maintain quiescence. Although it is unclear why there is an age-related upregulation of Fgf2 from the fiber, it has been suggested that it may be an inappropriate response by the muscle fiber attempting to induce repair mechanisms (Chakkalakal et al. 2012). Interestingly, it appears that blocking Fgf2 signaling at the satellite cell may have therapeutic benefits, preventing satellite cell loss (Chakkalakal et al. 2012). Satellite cells themselves may in fact contribute to the regulation of the extracellular matrix by directly influencing fibroblast function (Fry et al. 2014). Isolated satellite cells in vitro serve to negatively regulate key extracellular matrix mRNA expression in fibroblasts, suggesting a mechanism whereby satellite cells regulate their own microenvironment to ensure appropriate extracellular architecture (Fry et al. 2014). Importantly, a reduction in satellite cell number appears to lead to increased tissue fibrosis in aging muscle (Fry et al. 2015), contributing to age-related tissue fibrosis and dysfunction.
Other key muscle-derived cytokines and growth factors such as IL-6 and IGF-1 have been shown to change as a result of the aging process. IL-6 concentration in the circulation is increased in the elderly and thought to be associated with an age-related low-grade inflammation. Interestingly, elevated levels of IL-6 are associated with a loss of muscle strength (Visser et al. 2002; Pereira et al. 2009). Prolonged elevated levels of IL-6 were shown to induce muscle atrophy (Haddad et al. 2005) and thus may also negatively impact satellite cell function. IL-6 is a pleiotropic cytokine with divergent effects on many tissues depending on spatial and temporal changes in concentration (Pedersen and Febbraio 2008; Frost and Lang 2005; Kishimoto 2005). Chronically elevated systemic IL-6 is associated with a proinflammatory environment and muscle wasting conditions such as aging, sarcopenic obesity, and cancer cachexia (Roubenoff 1999, 2003). Animal studies using direct infusion of IL-6 into the circulation report that elevated IL-6 can induce a catabolic environment, promote muscle atrophy, and impair normal muscle growth (Haddad et al. 2005; Bodell et al. 2009). Human studies also illustrate strong associations between elevated serum IL-6, lower muscle strength, and reduced muscle mass associated with the aging process (Visser et al. 2002; Pereira et al. 2009
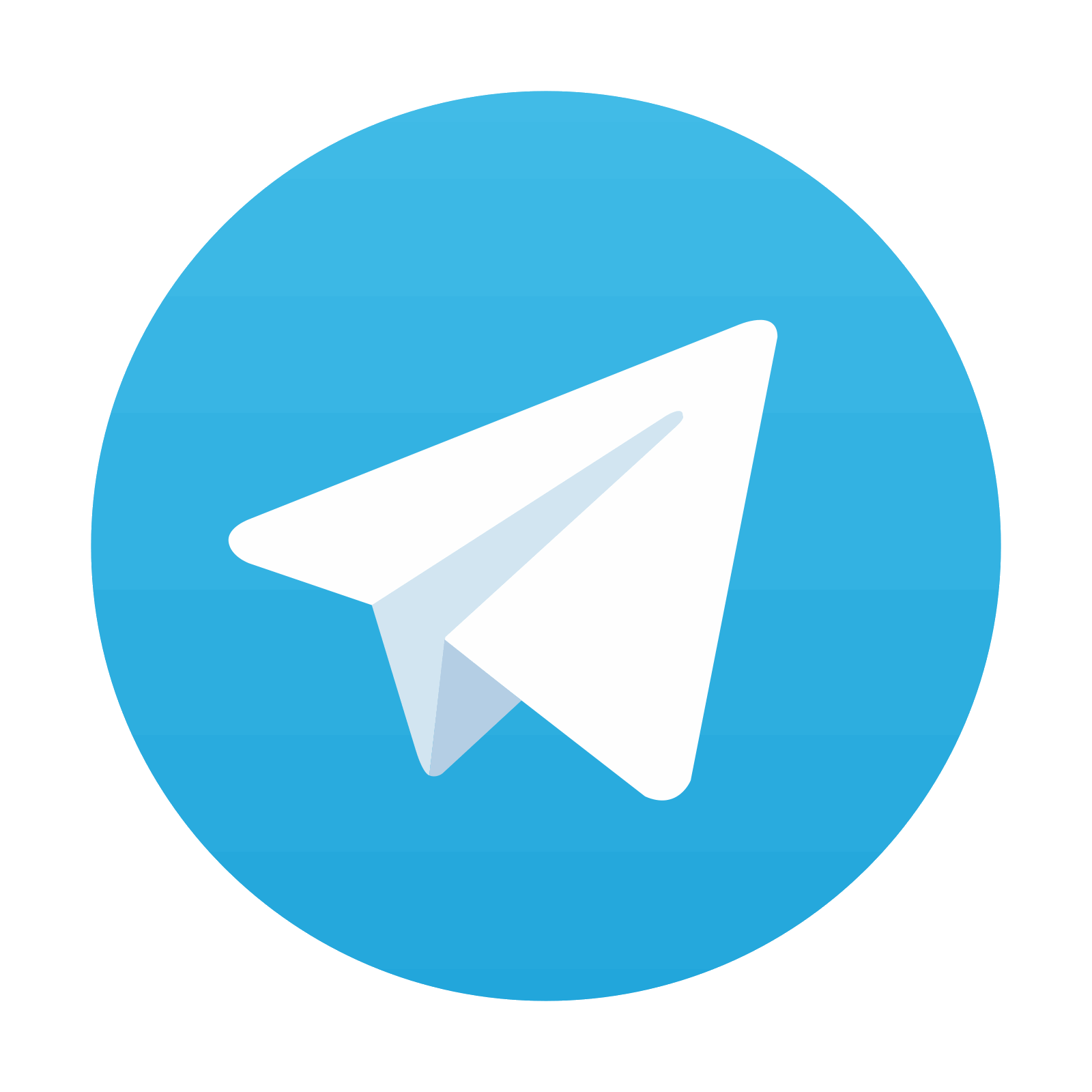
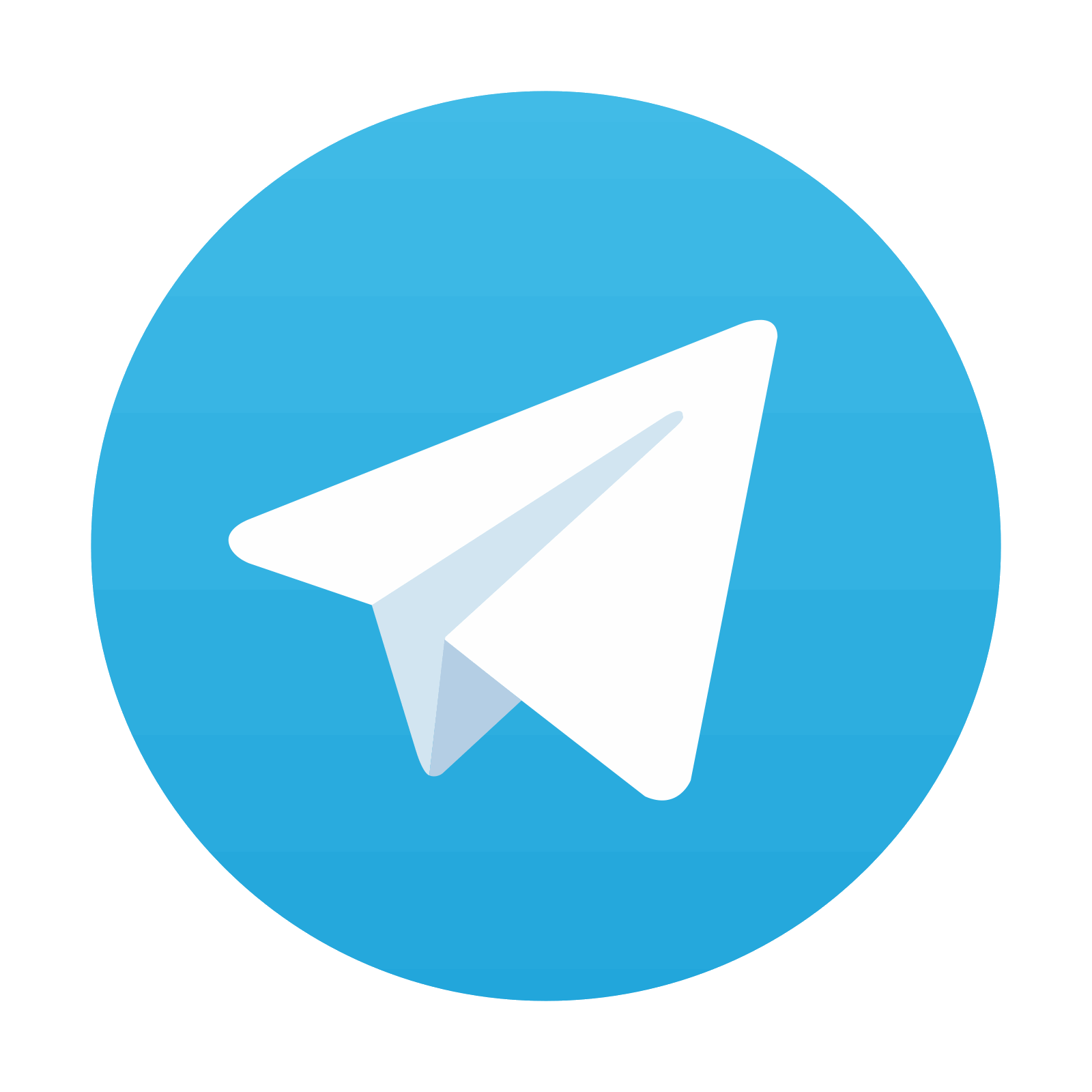
Stay updated, free articles. Join our Telegram channel
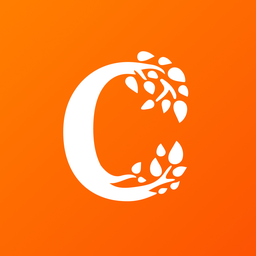
Full access? Get Clinical Tree
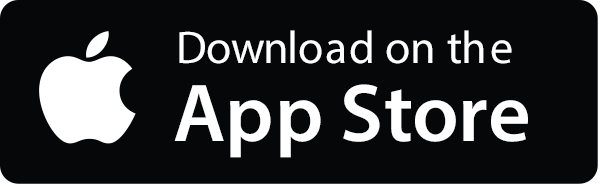
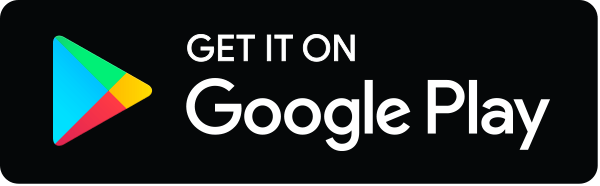