Fig. 6.1
Simplified representation of the hematopoietic hierarchy and the effects of aging (Adapted from Verovskaya and de Haan (2011)). HSCs residing in the bone marrow produce all the mature hematopoietic and immune cells that are found in the blood. The majority of HSCs resides in a quiescent G0 state (top left) and becomes activated into cycling and differentiation. During the gradual process of multilineage differentiation, HSCs lose the ability to self-renew (as indicated by round arrows) and become more committed to a certain lineage. In aged mice, HSCs have been found to more actively cycle. Moreover, hematopoiesis becomes skewed toward production of myeloid cells, while lymphoid production becomes deficient. Regeneration of red blood cells and platelets is also decreased with lifespan. Processes activated with age are indicated with red arrows, while decreased activity is depicted with blue arrows. LT-HSCs indicate long-term repopulating HSC, ST-HSCs short-term repopulating HSCs. Several described intermediate progenitor states are shown on the figure: MPP multipotent progenitor cells, LMPP lymphoid-primed multipotent progenitors, CMP common myeloid progenitors, CLP common lymphoid progenitors, MEP megakaryocyte-erythroid progenitors, GMP granulocyte-macrophage progenitors
Box 6.1
Hematopoietic stem cell – adult multipotent stem cell that is able to self-renew and differentiate into all mature types of immune and hematopoietic cells.
Functionally defined HSC – a cell that can support long-term robust multilineage repopulation of a host, whose own hematopoietic system was ablated (e.g., by irradiation). This definition is retrospective. Criteria for defining stemness differ from lab to lab and are based on either total donor cell presence (chimerism) in blood of transplant recipient or chimerism in specific mature blood populations. The time-scale for defining long-term repopulation varies but usually constitutes at least 4 months of activity after transplantation. A very common functional HSC definition is its ability to support production at least 1 % of myeloid and lymphoid cells for 4 months after transplantation.
Phenotypically defined HSC – hematopoietic cell expressing a panel of cell surface markers characteristic for HSCs. Phenotypic definitions of HSCs have become more and more stringent over the years, coinciding with discoveries of novel markers. Marker combinations that allow to achieve isolation of highly enriched HSC populations include among others:
CD150+ CD244− CD48− (SLAM) (Kiel et al. 2005)
CD45mid Rho− SP (Dykstra et al. 2007)
lineage− Sca1+ c-Kit+ (LSK) CD48− CD34− EPCR+ CD150+ (Dykstra et al. 2011)
CD45+ EPCR+ CD48− CD150+ (ESLAM) (Benz et al. 2012)
LSKCD34− CD150+ (Morita et al. 2010)
SP LSK (Challen et al. 2010)
CD45 is expressed on hematopoietic cells and allows to distinguish them from non-hematopoietic populations.
HSC clone – all progeny of a single HSC
Clone size – proportion of differentiated cells in blood generated by an HSC clone
Morphologically, HSCs cannot be discriminated from more differentiated progenitor cells (Fig. 6.1). However, a panel of cell surface markers, characteristically expressed on HSCs, has been identified. These include expression of Sca1, c-Kit, CD150 (one of the SLAM markers), and CD201 (EPCR) and lack of the expression of lineage surface markers CD48, CD244, Flt3, and CD34. Alternatively, the ability of HSCs to efflux certain dyes (Rhodamine-123 (Rho) and Hoechst 33342) can be also used for purification (Bertoncello et al. 1985; Wolf et al. 1993). This feature of HSC results from the expression of the ABC transporters P-glycoprotein and Abcg2 (Zhou et al. 2001). Hoechst 33342 exclusion results in a characteristic appearance of primitive cells during fluorescence-activated cell sorting (FACS), known as the “side population” (SP) (Goodell et al. 1996). A combination of multiple of these markers allows prospective isolation and characterization of HSCs with purity up to 50 %, defined by the ability of a single cell to reconstitute hematopoiesis of a lethally irradiated animal (Kiel et al. 2005; Dykstra et al. 2007, 2011; Challen et al. 2009; Kent et al. 2009).
Aging has been shown to increase both the relative frequency and the absolute number of HSCs in the bone marrow of C57BL/6 mice. Initial studies using unfractionated bone marrow cells had demonstrated a competitive advantage of old cells when co-transplanted with equal numbers of young cells (Harrison 1983), which was attributed to higher concentrations of repopulating cells in the aged population (Harrison et al. 1989). More recent studies, using different combinations of phenotypic markers, confirmed a remarkable increase in the pool size of HSC upon aging (Dykstra et al. 2011; Morrison et al. 1996; Rossi et al. 2005; Sudo et al. 2000). An example of such a phenotypic increase of primitive hematopoietic population upon aging is shown in Fig. 6.2. The extent of such expansion was seven- to 16-fold (Dykstra and de Haan 2008). Furthermore, while the frequency of HSCs is similar among individual young mice, HSC frequencies become highly variable in old mice, indicating a possible loss of control of HSC pool size with age (Dykstra et al. 2011).
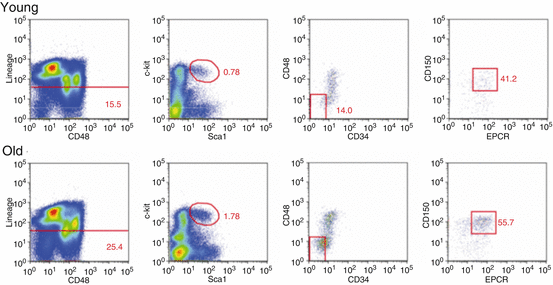
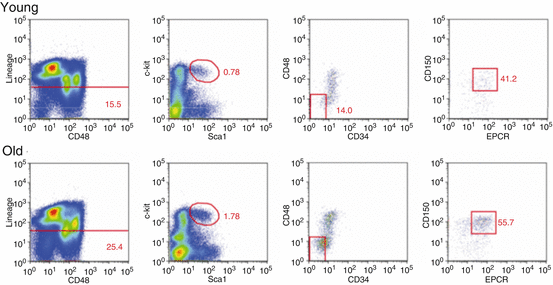
Fig. 6.2
Number of phenotypically defined HSCs increases with age. Here we show the gating strategy for isolation of LSK CD48− CD150+ EPCR+ CD34− cells (of young ~4-month-old and 1 of ~25-month-old animals) that have robust repopulating potential in vivo (Dykstra et al. 2011). Note that percentages of primitive cells are higher for an old mouse at all gating stages
Despite many advances that have been made in the identification of the phenotype of HSC, “stemness” of a (murine) HSC can only be demonstrated retrospectively by its ability for robust multilineage repopulation of a myeloablated host. An HSC is often defined by the ability of a cell to support at least 1 % lymphomyeloid chimerism in a recipient animal for 4 months posttransplantation (Ema et al. 2005; Miller and Eaves 1997). Primitive cells contributing to peripheral blood cells less than 1 % are usually unable to sustain robust blood production in the long-term or upon secondary transplantation. Therefore, they are not considered bona fide stem cells. In contrast to studies where whole bone marrow cells were used, transplantations of purified aged HSCs demonstrated a two- to sevenfold decrease in functional stem cell frequency in vivo (Dykstra et al. 2011; Morrison et al. 1996; Rossi et al. 2005; Yilmaz et al. 2006). Moreover, compared to young, aged HSCs have been shown to exhaust more rapidly upon serial transplantation (Kamminga et al. 2005). Finally, the ability of HSCs to generate cells of all different lineages changes as a function of age and switches toward production of myeloid cells concurrent with decreased lymphopoiesis (Morrison et al. 1996; Sudo et al. 2000; Liang et al. 2005) (indicated in Fig. 6.1).
6.3 Not All Stem Cells Are Equal
Historically, it has been assumed that the HSC pool is homogeneous and all HSCs are uniform in their ability to produce cells of all lineages (as shown in Fig. 6.1). However, this concept was challenged by clonal studies demonstrating profound heterogeneity among HSCs regarding three of their key properties: (1) repopulation potential, (2) cycling activity, and (3) developmental program.
It was shown that individual HSCs display a marked diverse and unpredictable self-renewal capacity and repopulating activity upon transplantation into irradiated host mice (Dykstra et al. 2007; Ema et al. 2005). For instance, upon single-cell transplantation, purified cells can generate highly variable numbers of circulating white blood cells ranging from 1 to >80 % chimerism (Dykstra et al. 2007; Ema et al. 2005).
Further, the HSCs are highly heterogeneous in regard to their cycling rate (Wilson et al. 2008; Foudi et al. 2009). While HSCs have a high proliferative capacity, their turnover is slow, what protects HSCs from accumulating mutations and prevents their exhaustion (Dykstra et al. 2006; Orford and Scadden 2008; Arai and Suda 2007). Analysis of cell cycle in the primitive compartment has shown that more than 70 % of phenotypically defined HSCs are found in quiescent G0 stage, opposed to less than 10 % of progenitor population (Wilson et al. 2008). Multiple other studies supported this notion (Wilson et al. 2008; Passegue et al. 2005; Cheshier et al. 1999). Several reports suggest presence of at least two populations within the HSC pool – “homeostatic” HSCs that have a turnover time of approximately 1 month and “dormant” HSCs that are entering the cell cycle once in around 170 days (Wilson et al. 2008; Foudi et al. 2009).
Moreover, a highly variable ability of HSCs to produce myeloid and lymphoid progeny is well documented. This was first reported in retroviral marking studies where different vector integration profiles were observed in myeloid and lymphoid tissues (Jordan and Lemischka 1990). Later, experiments in animals transplanted with limiting dilutions of bone marrow cells (Muller-Sieburg et al. 2002) and in single-cell transplant recipients (Dykstra et al. 2007) demonstrated the presence of at least three distinct developmental types of behavior of HSCs. These studies established that individual cells can preferentially support myeloid cell production (so-called α or myeloid-biased HSCs) and lymphoid cell development (γ or lymphoid-biased HSCs) or be equally prone to generate both lineages (β or balanced HSCs). High expression of CD150 has been demonstrated to correlate with a myeloid potential of HSCs (Beerman et al. 2010a). Lymphoid-biased HSCs have been shown to have a relatively short lifespan and to exhaust readily upon secondary transplantation (Muller-Sieburg et al. 2004). On the contrary, myeloid-biased HSCs had the longest lifespan and the highest self-renewal capacity of all HSC types (Muller-Sieburg et al. 2004).
Distinct gene expression profiles appear characteristic to HSCs with distinct developmental programs. CD150 (SLAM) and SP profiling in phenotypically isolated HSCs allowed their sub-fractionation into lymphoid-biased and myeloid-biased subsets (Challen et al. 2010) (Box 6.1). Serial transplantation experiments revealed that a lower SPLSK CD150high population preferentially contributed to LT-HSC, MPP, and CMPs (Fig. 6.1), while upper SPLSK CD150low cells produced significantly more CLPs. Microarray analysis carried out on those two populations revealed that pathways involved in cell cycling and metabolism were highly activated in lymphoid-biased HSCs (Challen et al. 2010).
During aging, the proportion of myeloid-biased HSCs in the bone marrow increases at the expense of lymphoid-biased and balanced HSCs (Challen et al. 2010; Beerman et al. 2010b; Cho et al. 2008; Morita et al. 2010). Such an expansion of myeloid-primed HSCs is likely to result from a combination of higher self-renewal potential (Beerman et al. 2010b), different response to cytokines, and lower metabolic activity (Challen et al. 2010) of this population. Moreover, while the pool of young HSCs is largely quiescent, a substantially higher fraction of aged HSCs is cycling (Morrison et al. 1996; Sudo et al. 2000; Yilmaz et al. 2006; Takizawa et al. 2011) (Fig. 6.1).
6.4 Changes in Homing and Migration of Stem Cells Upon Aging
The ability to engraft bone marrow and to migrate throughout the body is an essential property of HSCs that allows them to exit the fetal liver and repopulate bone marrow in the initial stages of development. Studies in the 1960s demonstrated that a small number of repopulating cells is also present in the blood of mice in steady-state conditions (Goodman and Hodgson 1962). These circulating cells are capable of reentering available HSC niches, as demonstrated by experiments with pairs of parabiotic mice that share their circulation (Wright et al. 2001) and by transplantation of bone marrow cells into nonirradiated recipients (Ramshaw et al. 1995). Homing of cells to myeloablated or unmanipulated niches is a prerequisite in experimental or clinical bone marrow transplantations, where intravenous cell injection is sufficient for grafting and regeneration of the hematopoietic system (van Os et al. 2010).
Several studies demonstrated that upon aging, the engraftment and homing ability of HSCs decreases ~2-fold. Liang et al. demonstrated diminished short-term (24 h) homing of old HSCs by limiting-dilution competitive transplantation of young and aged HSCs (Liang et al. 2005). Recently, our lab confirmed this observation by direct measurement of fluorescent cells in bone marrow after co-transplantation of HSCs isolated from old and young differentially colored transgenic donors (Dykstra et al. 2011).
On the contrary, levels of both mobilized progenitors and transplantable HSCs were ~5-fold higher in peripheral blood and spleen of granulocyte colony-stimulating factor (G-CSF)-treated 25-month-old mice compared to young 3-month-old animals (Xing et al. 2006). Xing et al. proposed that lower adhesion of aged HSCs to stromal cells and increased activity of small Rho GTPases that are involved in regulation thereof play a role in this change (Xing et al. 2006).
Interestingly, data generated in our lab demonstrates that on a clonal level young and aged HSCs are similar in their migratory behavior (Verovskaya et al. 2014). When purified HSCs were labeled with DNA barcodes and transplanted into irradiated hosts, barcoded clones of both young and old HSCs were unequally distributed across the skeleton. However, administration of G-CSF led to redistribution of HSC around the skeleton. At the same time, clonal analysis demonstrated that the pool of old HSCs was composed of multiple small clones, whereas the young HSC pool contained fewer, but larger clones (Verovskaya et al. 2013).
The observed functional decline in aged HSCs is reflected in their transcriptome and epigenome, which will be discussed in the next section. A final issue that we will discuss is how HSCs reside in an hypoxic environment and how oxidative metabolism, production of reactive oxygen species (ROS), and DNA repair pathways are linked to diminished self-renewal and proliferative abilities of old HSCs.
6.5 Gene Expression Changes
The increased number of HSCs in aged animals, accompanied by defects in their repopulating ability, as discussed above, suggests that changes in the transcriptional and epigenetic landscape occur with time. However, data describing the transcriptome of old HSCs are limited (Rossi et al. 2005; Chambers et al. 2007a; Noda et al. 2009; Sun et al. 2014) and highly variable. Our understanding of the epigenome is even more restricted, with only two studies recently been published (Beerman et al. 2013; Sun et al. 2014). In this section, we will discuss molecular mechanisms of HSC aging identified thus far.
To date, three groups have independently used microarray analysis to compare gene expression profile in young and aged HSCs. Categories of genes that were upregulated with age in HSCs included those involved in NO-mediated signal transduction, stress response (protein folding), and the inflammatory response. Age-dependent repressed genes were enriched for those involved in the preservation of genomic integrity, e.g., chromatin remodeling and DNA repair (Rossi et al. 2005; Chambers et al. 2007b). Surprisingly, while results of all studies indicate that hundreds of genes are differentially expressed, only five of those genes were overlapping among these reports. Of note, all of these studies used pooled samples from multiple mice to initiate the analysis. While differences in the panel of markers used for selection of HSCs (Box 6.1) could contribute to such discrepancies, it is also likely that the pronounced heterogeneity of individual aged mice that we discussed above can explain these differences. In the future, analysis of individual mice will be instrumental for answering this question. Nevertheless, below we will further discuss molecular players that were indicated in those and other studies.
Genes that have been found to be involved in HSC aging thus far include receptors, chromatin modifiers, transcription factors, and genes involved in the DNA damage response and cell proliferation. Two of the highly upregulated genes involve the surface adhesion molecules P-selectin and intercellular adhesion molecule 1 (ICAM1) (Fig. 6.3) (Rossi et al. 2005; Chambers et al. 2007a). Increased levels of P-selectin and ICAM1 are implicated in a higher mobility of those cells and can interfere with HSCs engraftment in bone marrow, which is strictly dependent on adhesion. Of these genes, P-selectin has been shown to serve as a marker for physiological stress, including inflammation, and it is therefore interesting that it has now been shown to be activated upon aging. It mediates the leukocyte-vascular endothelium interaction involved in leukocyte extravasation and therefore appears important for HSCs migration (Karin and Ben-Neriah 2000). At the same time, a decreased expression level of chromatin modifiers (SWI/SNF and PRC2 core members) implicates reorganization of the chromatin structure (Chambers et al. 2007a; Beerman et al. 2013; Hidalgo et al. 2012). Epigenetic perturbations may propagate skewing toward the myeloid lineage and be involved in anemia in aged individuals, because of hypermethylation of promoter regions of genes regulating lymphopoiesis and erythropoiesis (Beerman et al. 2013). Further, aged HSCs are likely to display a more euchromatic genome state, since genes encoding chromatin compaction tend to be downregulated in old HSCs (besides the abovementioned SWI/SNF remodelers, expression of related chromatin remodeling genes such as Smarca4 and Smarca1, as well as histone deacetylases Hdac-1, Hdac-5, and Hdac-6 and a DNA methyltransferase Dnmt3b is decreased) (Chambers et al. 2007a).
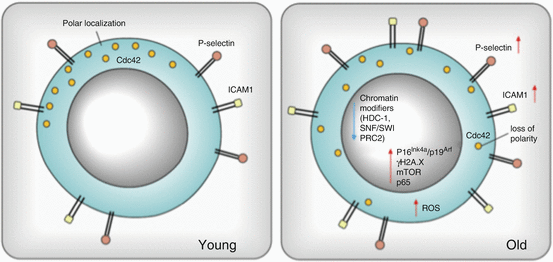
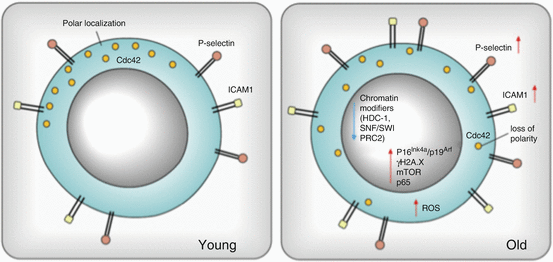
Fig. 6.3
Molecular mechanisms of murine HSC aging. The figure summarizes molecules/pathways reported to be changed in aged HSCs. Increased expression is shown with red arrows and decreased expression with blue. Changes in cellular localization of Cdc42 are depicted: while in young cells it is polarly distributed, in old HSC it is apolar
Moreover, recent RNA-Seq-based report on epigenomic state of old HSCs documents an increased occurrence of the transcription activating mark-H3K4me3 across self-renewal genes and decreased methylation of HSC maintenance genes combined with hypermethylation of gene promoters associated with differentiation, reflecting phenotypical HSC aging (Sun et al. 2014). Interestingly ribosomal biogenesis seems to be a particular target of aging with hypomethylation of rRNA genes.
The expression of P-selectin, one of the few proteins to be consistently upregulated in aged HSCs, is regulated by NF-κB (the p65 isoform). In HSCs from aged mice, it has been observed that p65 accumulates in the nucleus, indicating transcriptional activation. Increased abundance of this protein in the nucleus of aged HSC may induce upregulation of Toll-like receptor 4 (TLR-4), as well as higher levels of proinflammatory molecules in the plasma of aged individuals (Bruunsgaard et al. 1999; Ershler and Keller 2000). Such higher levels of proinflammatory cytokines (TNFα, IL-1β), together with increased expression of inflammation-related genes, support the concept of inflammation playing a significant role in the aging process (Chambers et al. 2007a).
Two other genes that have often been associated with the aging process are p16 Ink4a and p19Arf, which are tumor suppressors that induce loss of HSCs without inducing apoptosis (Ito et al. 2004). Furthermore, p16 Ink4a has been proposed as a biomarker of aging as its expression increases with age in mammalian tissues, including murine bone marrow (Krishnamurthy et al. 2004). The presence of p16Ink4a restricts the number of HSCs and affects their serial repopulation capacity, cell cycling, and apoptosis (Janzen et al. 2006). However, a causal role for p16Ink4a in the aging process is still under discussion since an effective repression of this gene by H3K27me3 mark in young and old HSCs was recently reported (Sun et al. 2014). Moreover, in the study from Attema et al., no increased levels of p16Ink4a transcripts in individual aged HSCs were found (Attema et al. 2009). The expression of p16Ink4a and p19Arf is mediated by the activity of Polycomb Repressive Complex 2 (PRC2). Recent studies showed upregulation of PRC2 core component in aged HSCs, suggesting in fact increased repression of this locus upon aging (Hidalgo et al. 2012; Norddahl et al. 2011). This is in line with findings that have shown hypermethylation of loci targeted by PRC2 during aging (Beerman et al. 2013).
Aside from the possible, if not likely, contribution of epigenetic mechanisms underlying stem cell aging, there are also many studies that indicate a causal role of genetic and genomic alterations in the aging process. A decline in the expression of genes involved in DNA repair, resulting in an increased rate of non-repaired DNA damage, is thought to be a driving factor in aging. The role of these pathways is described in the next section.
6.6 The Role of Oxidative Metabolism and DNA Repair in HSC Aging
HSCs reside in the bone marrow and are supposed to replenish blood cell production for the entire lifetime of the organism. Both cell-extrinsic localization and cell-intrinsic DNA damage repair mechanisms allow HSCs to maintain their functionality. HSCs appear not be lodging randomly in the bone marrow, but in fact preferentially reside in a low oxygen environment which: (1) reduces molecular damage that results from oxidation by ROS, (2) determines their energy status, (3) induces pathways that are active under hypoxic conditions, and (4) decreases the rate by which DNA mutations accumulate. Apart from preventing damage by avoiding exposure to oxygen, HSCs implement efficient mechanisms of DNA repair to remove deleterious mutations and prevent these to propagate to their progeny. To accomplish this ability, HSCs can employ two independent pathways, depending on the type of the DNA damage and their cell cycle status. We will discuss these parameters in more detail in this section.
One of the first concepts suggesting a link between ROS and the aging process is the “free radical theory of aging,” proposed by Harman in the 1950s (Harman 1956). This theory argues that the effect of ROS on cellular components (proteins, nucleic acids, and lipids) may be key in determining the lifespan of the organism. Thus, if we consider that the lifespan of the organism is at least partially determined by the activity of stem cells to maintain tissue homeostasis, effective neutralization of ROS may be crucial for maintaining the stem cell pool. Indeed, several recent studies have demonstrated a role of ROS, and how managing ROS levels and, more general, metabolism affect proper HSC functioning.
The redox potential in HSCs is strictly regulated by intrinsic and extrinsic factors that will be described later in detail. The average O2 concentration in BM is approximately three times lower compared to blood (Klebanov et al. 2000; Parmar et al. 2007). This suggests that HSCs are residing in an environment with limited availability of oxygen, which propagates glycolytic metabolism (Simsek et al. 2010). However, the content of mitochondria in HSC is relatively high compared to myeloid cells, which suggest that they are less active (Norddahl et al. 2011).
Although the net production from 2 glucose molecules is only 2 ATP (versus 36–38 ATPs during mitochondrial oxidation), this metabolic landscape is compatible with the low-energy requirements of HSC (Folmes et al. 2012) and significantly reduces the rate of ROS production. Whether ROS levels in HSCs are mainly determined by the niche location or by the stem cell-intrinsic activation status remains to be further investigated.
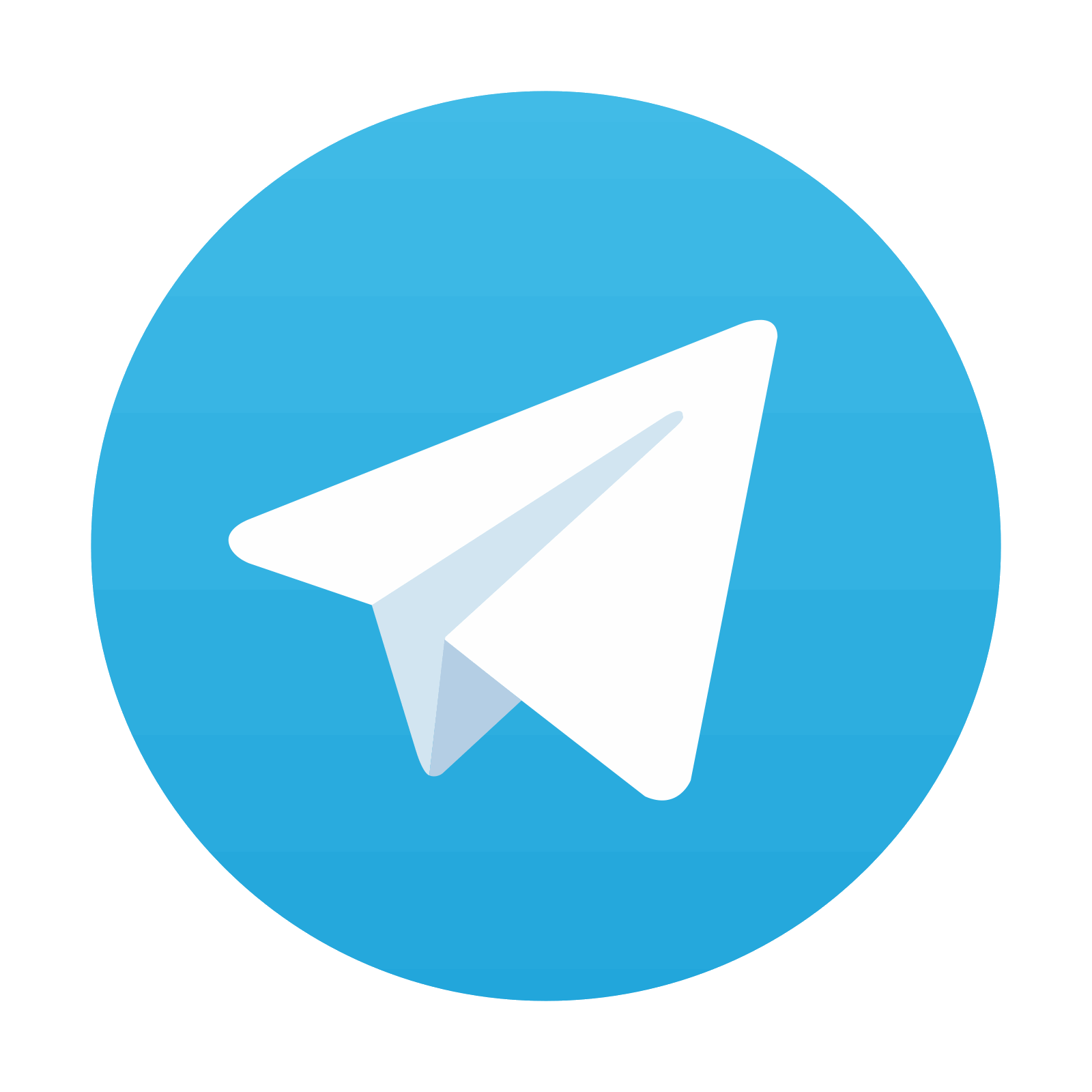
Stay updated, free articles. Join our Telegram channel
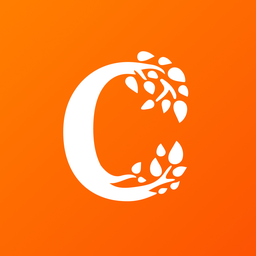
Full access? Get Clinical Tree
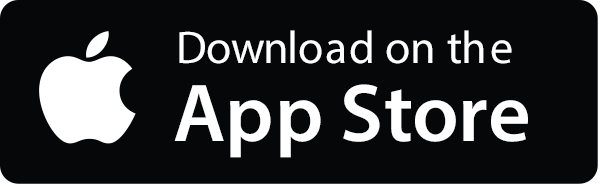
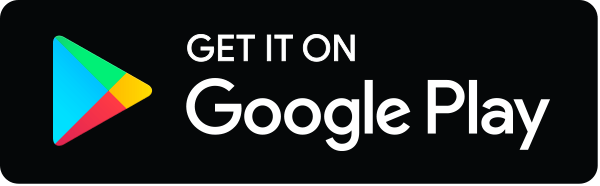