Energy Expenditure
Within the context of a highly controlled in-patient environment in which subjects are maintained at stable body weights by being fed a liquid formula before and after weight loss, we have found that maintenance of a 10 % or greater reduction in body weight in lean or obese individuals is accompanied by an approximate 20–25 % decline in 24-h energy expenditure (TEE) [45, 61]. This decrease in weight maintenance calories is 10–15 % below what is predicted solely on the basis of alterations in weight and body composition [45, 61]. Thus, a weight-reduced individual will require ~300–400 fewer calories per day to maintain the same body weight and level of physical activity as a never-obese individual of the same body weight and composition. The ~300–400 kcal magnitude of adaptive thermogenesis reported in in-patient studies of weight-reduced individuals [45] is remarkably similar to the changes in energy balance (increased physical activity and decreased energy intake) reported by subjects in the National Weight Control Registry [39]. This adaptive thermogenesis occurs regardless of whether the person is initially lean or obese [45]. It should be noted that this response is heterogeneous, for example, the range of decline in energy expenditure per unit of fat-free mass in 45 individuals studied before and after a 10 % weight loss ranged from −38 % to −6 % (unpublished data based on [45]). It should also be noted that while there is additional adaptive thermogenesis following additional weight loss, it appears that the majority of the relative hypometabolism is induced early on in the weight loss process [62] and that the declines in energy expenditure following a weight loss of 10 % from usual weight are greater than those following additional weight loss from 10 % to 20 % below usual [45].
The necessity for these long-term changes in lifestyle is consistent with the observation that the reduction in 24-hour energy expenditure (TEE) persists in subjects who have sustained weight loss for extended periods of time (6 months–7 years) in circumstances of enforced caloric restriction in the biosphere 2 project [63] and lifestyle modification [64]. Analyses of data regarding energy expenditure in bariatric surgery patients are often confounded by the effects of the surgery on the co-morbidities and medications that preceded the procedure. That said, some studies have reported similar declines in energy expenditure following bariatric surgery to those seen following dietary weight reduction [65], while others have suggested that the effects weight loss via bariatric surgery on energy expenditure may be “blunted” compared to dietary weight loss [66–69].
TEE is the sum of resting energy expenditure (REE; cardiorespiratory work and the work of maintaining transmembrane ion gradients at rest; approximately 60 % of TEE), the thermic effect of feeding (TEF; the work of digestion; approximately 5–10 % of TEE), and non-resting energy expenditure (NREE, energy above resting that is expended in physical activity; approximately 30–40 % of TEE in sedentary individuals). Each of these components of TEE is differentially affected by weight reduction. There is no significant change in TEF (the fraction of the energy contained in food that is utilized to digest it) following weight loss [45]. REE per unit of metabolic mass has been reported to show no change [70–72] or a moderate decrease accounting for about 25–35 % of the decline in TEE beyond that predicted on the basis of body composition changes [45, 61, 73]. The variability in results probably reflects inter-study differences in multiple factors including degree and duration of weight stability before and after weight loss, ambient temperature, and changes in subject fitness and time spent in physical activity following weight loss [74]. In contrast, during dynamic weight loss there is little or no debate that REE is significantly lower (approximately 15–25 %) than at usual weight [45, 75–79]. Regardless of whether or not there are significant declines in REE following weight loss, NREE is clearly the compartment of energy expenditure that is most affected by changes in body weight [45, 76, 80, 81] consistent with the importance of physical exercise in the successful maintenance of reduced weight [39, 40].
The pre-eminence of NREE—accounting for over 70 % of the variance in the decline in TEE below predicted values in weight-reduced subjects [80, 82]—could be due to declines in the actual amount of physical activity or increased contractile efficiency of skeletal muscle, or both. In studies of rodents and of out-patient humans, maintenance of a reduced body weight is associated with no change or an increase, rather than decrease, in the amount of time spent in physical activity [45, 72, 76, 78, 83], supporting the view that skeletal muscle work efficiency is increased [80, 81] following weight loss. These effects are most evident at low levels of work/power (10–25 watts during bicycle ergometry) suggesting that some of the muscle-mediated opposition to reduced weight maintenance might be diminished by exercising at higher levels of power output [80, 84] or by engaging in exercises such as resistance training which favor increased expression of more powerful but less efficient myosin heavy chain isoforms (see below) [85, 86].
Studies of skeletal muscle chemomechanical efficiency (calories expended above resting per unit of power generated) in weight-reduced subjects indicate that maintenance of a reduced body weight is associated with an approximate 20 % increase in skeletal muscle work efficiency and an approximate 18 % relative increase in the fractional use of free fatty acids as fuel during low level exercise [52, 80], whether measured by bicycle ergometry or 31P-NMR muscle spectroscopy [80]. These results are consistent with vastus lateralis muscle biopsies in which the ratio of glycolytic (phosphofructokinase, PFK) to oxidative (cytochrome oxidase) enzyme activities is significantly decreased and the expression of the more efficient myosin heavy chain (MHC) and sarcoplasmic endoplasmic reticulum Ca2+-dependent ATPase (SERCA) isoforms (MHCI and SERCA2) are significantly increased following weight loss [52, 53, 80]. The magnitude of these changes in muscle efficiency, biochemistry, and gene expression is potentially physiologically sufficient to account for the increased skeletal muscle efficiency and decreased utilization of glucose as fuel during low level exercise following weight loss [52, 53, 80].
Neuroendocrine Function
By virtue of its constituent neuronal outflow tracts to the ANS, neuroendocrine axes, and cortical tracts subserving food intake and energy expenditure, the hypothalamic pro-opiomelanocortin (POMC)-melanocortin-melanocortin 4 receptor (MC4R) pathway provides a central nexus for the integrated effects on energy intake and expenditure of hypoleptinemia or weight loss [87, 88]. Briefly, POMC is cleaved to alpha-melanocyte stimulating hormone (α-MSH) and beta-endorphin (β-EP) as well as other bioactive molecules. Alpha-MSH stimulates release of hypothalamic pro-TRH. β-EP inhibits the release of hypothalamic corticotropin releasing factor (CRF; an anorexiant neuropeptide). POMC expression is sensitive to ambient leptin concentrations and therefore is decreased in low leptin states, such as congenital leptin or leptin receptor deficiency or during and following weight loss [89, 90] with resultant expected increased activity of the hypothalamic–pituitary–adrenal (HPA) axis and decreased activity of the hypothalamic–pituitary–thyroid (HPT) axis. If the weight loss or hypoleptinemia is sufficiently severe, there is also a functionally significant decreased activity of the hypothalamic–pituitary–gonadal (HPG) axis resulting in infertility (and protection of the female from conceiving in times of undernutrition with neither the mother, the offspring, or their genes are likely to survive the pregnancy or early feeding period) [47].
The importance of the HPA axis in energy homeostasis is exemplified by the observation that the hyperphagic, hypometabolic (similar to weight-reduced humans), and hypercortisolemic phenotypes of leptin-deficient or leptin-resistant rodents are abolished by chemical or surgical adrenalectomy [91]. Hypercortisolemia results in loss of lean body mass and increased partitioning of stored calories to fat [88]. Studies of the HPA axis in which human subjects were assessed following various weight loss regimens have reported increases [92], decreases [93], and no change [94] in indices of cortisol production following weight loss. Discrepancies among such studies may reflect differences in subject populations regarding exercise, gender, age, or weight loss regimens, as well as the degree of weight stability at the time of study.
Thyroid hormone increases energy expenditure by increasing heart rate, blood pressure, muscle ATP consumption (largely by stimulating production of muscle ATPase and favoring expression of the less mechanically efficient more glycolytic myosin heavy chain II (MHCII) isoform) [53, 95]. The thyroid-hormone-deficient patient is hypotensive, bradycardic, and lethargic and tends to gain weight while the hyperthyroid patient is hypertensive and tachycardic and tends to lose weight [96, 97]. Both weight loss and the maintenance of a reduced body weight are associated with small but statistically significant decreases in circulating concentrations of triiodothyronine (T3) and increases in the circulating concentrations of its bioinactive enantiomer reverse T3 (rT3) [46, 51], suggesting that weight loss results in increased peripheral conversion of thyroxine (T4) to rT3 [46]. Thyroid releasing hormone (TRH)-stimulated pituitary thyroid stimulating hormone (TSH) release is not diminished either during caloric restriction [98] or after weight loss [99] in humans. However, as discussed above, low ambient leptin reduces POMC production in hypothalamic neurons resulting in decreased activity of hypothalamic pro-thyroid releasing hormone (pro-TRH) neurons in rats [100] as a result of decreased α-MSH [101]. Therefore, the decline in TSH during and after weight loss [46, 51] may reflect decreased production of TRH rather than decreased sensitivity of TSH neurons.
Autonomic Nervous System Function
The autonomic nervous system includes major outflow tracts linking afferent biochemical signals regarding energy stores and efferent tracts regulating energy homeostasis. Increased parasympathetic nervous system (PNS) tone slows heart rate and decreases resting energy expenditure. Sympathetic nervous system (SNS) tone modulates feeding behavior via effects on various gut peptides and transmission of nutrient-derived signals to the brainstem and also mediates the effects of cannabinoid receptor-1 activity [102, 103]. SNS tone also directly increases heart rate, and acts directly on the thyroid gland to increase secretion of thyroid hormone [48, 104] (24 h urinary norepinephrine excretion accounts for a significant proportion of the variance in energy expenditure and its subcomponents in weight stable subjects [46]).
The maintenance of a reduced body weight is associated with significant declines in SNS tone and increases in PNS tone [46, 48, 105] which may account for a significant fraction of the hypometabolic state through direct effects on skeletal muscle, and/or indirectly via effects on circulating concentrations of thyroid hormones [46, 106, 107]. Thus, weight-loss-mediated changes in autonomic nervous system activity may constitute a link between weight-loss-associated changes in energy and neuroendocrine homeostasis.
Brown Adipose Tissue
Brown adipose tissue (BAT) allows the uncoupling of mitochondrial substrate oxidation from ATP production and release some of the energy of fatty acid oxidation as heat [108]. BAT is a major contributor to adaptive thermogenesis in small mammals [109] via its role in both obligatory (maintenance of body temperature) and facultative (response to low ambient temperature) thermogenesis [110]. Physiologically, BAT activation and subsequent heat generation depend upon the integration of input from the SNS activation of adrenoreceptors (predominantly β3) [111], with activation of at least one of the thyroid hormone receptor (TR) subtypes (TRα or TRβ) [110]. Since both SNS tone and circulating concentrations of bioactive thyroid hormones are reduced following weight loss (see above), it is possible that a significant fraction of the unexplained variance in energy expenditure following weight loss is attributable to changes in the BAT [112].
Recent advances in positive emission tomography (PET) scanning technology have allowed detailed imaging of BAT using uptake of 2-[18F]fluoro-2-desoxy-glucose (FDG) and a hybrid scanner. Several groups have demonstrated the ability to detect BAT in healthy human beings with varying results as to whether thermal stimuli are necessary for its detection [113–115].
However, while BAT is a major contributor to adaptive thermogenesis in small mammals [109], and contributes to non-shivering thermogenesis in human infants, its thermogenic role in adult humans remains unclear. Previous studies showed a lack of a significant presence of BAT in humans except under extreme conditions of hypercatecholaminemia [116, 117] and, until recently, quantitative assessment of the contribution of BAT to total adaptive thermogenesis in humans has not been performed and was probably underestimated. Recent studies of cold-induced BAT thermogenesis in humans who were placed in a suit perfused with 18 °C water showed an activation of an average of ~168 ml of BAT (vs. no detectable BAT at room temperature of 25 °C) and an average increase in TEE of ~77 kcal/h (1,857 kcal/day, 11 kcal/ml BAT/day) [118]. Based on these data, the sustained activation of approximately 30 ml of BAT would be sufficient to reverse most of the adaptive thermogenesis that occurs during maintenance of reduced body weight [45]. Since no BAT activation was detected at room temperature, it is likely that whatever role BAT plays in human energy homeostasis beyond the neonatal period is likely to more evident in obligatory (metabolic) than in facultative (thermoregulatory) thermogenesis. Clothing, central heating, heated transportation ensure that in developed countries most individuals spend most of their time in thermoneutral conditions, reducing the need for facultative thermogenesis and possibly contributing to the increasing prevalence of obesity [11, 119]. The recent identification of mechanisms that could potentially result in the “browning” of white adipose tissue (WAT), thus increasing energy expenditure by WAT, may provide a mechanism for increased functional importance of BAT-like cells in humans [120].
Energy Intake (See Table 7.1)
Table 7.1
Effects of weight loss and leptin on fMRI response to visual food cues
Brain areas more active in response to visual food cues at 10 % reduced weight (leptin-depleted) than in leptin-sufficient states (usual weight or weight-reduced but with leptin repletion) | Brain areas less active in response to visual food cues at 10 % reduced weight (leptin-depleted) than in leptin-sufficient states (usual weight or weight-reduced but with leptin repletion) | ||
---|---|---|---|
Structure | Net function (Effect) | Structure | Net function (Effect) |
Brainstem | ↑ Signal processing (food recognition) | Hypothalamus | ↓ Integration of leptin/humoral signaling |
Globus pallidus | ↑Food reward | Amygdala | ↓ Response to sensory cues (feelings of fullness) |
Insula | ↑Food reward expectation | Cingulate | ↓ Self-control and error recognition (dietary restraint) |
Ventral striatum | ↑ Food reward and motivation | Inferior parietal lobule | ↓ Response based on experience (action based on previous knowledge) |
Lingual and superior temporal gyri | ↑ Affective response to high and low caloric density foods | ||
Net effect after weight loss | ↑ Food reward | Net effect after weight loss | ↓ Food restraint |
As discussed above, the long-term constancy of body weight despite large day-to-day variations in caloric intake and physical activity indicates that, at usual weight, energy intake and energy output are “coupled” and, over time, vary directly with each other thus maintaining a relative constancy body energy stores. If this coupling persisted following weight reduction then weight-reduced individuals would naturally eat less in response to the hypometabolic state induced following weight reduction. In this physiological scenario, it would be relatively easy for a weight-reduced person to comply with dietary recommendations to sustain weight loss even if the number of calories required to sustain the weight reduction were substantially lower than someone naturally at the same weight.
Unfortunately for those attempting to lose weight and to sustain the loss, this “coupling”—which reduces caloric intake in response to decreased energy expenditure—is disrupted during and following weight loss [121]. During dynamic weight loss, i.e., in a state of negative energy balance, and during maintenance of reduced body weight (i.e., a state of energy balance), human beings and rodents are both hungrier (willing to eat more often) and less satiated (willing to eat more per meal) [54, 122]. Briefly, during strict maintenance of a reduced body weight on a bland liquid formula diet with little or no hedonic value, functional magnetic resonance imaging (fMRI) studies of overweight or obese subjects before and after weight loss demonstrate increased blood oxygen level-dependent (BOLD) signaling in response to food in the orbitofrontal cortex and brain areas mediating reward [55]. They also demonstrate decreased BOLD signaling in response to food in the hypothalamus and in the pre-frontal cortex and brain areas mediating restraint. These changes in neural signaling in response to food following weight loss are consistent with the observation that before a liquid formula meal, these same weight stable subjects report feeling less full (hungrier, greater food reward expectation) and perceive themselves as having eaten less (despite ingesting the same 300 kcal 2 h before the study) and also perceive themselves as less satiated and having eaten less despite having increased their actual intake (diminished food restraint) following ad libitum formula consumption [54]. The simultaneous declines in both energy expenditure and satiety following weight loss conspire to create the optimal biological circumstance for weight regain [123]. Interestingly, studies of subjects in the National Weight Control Registry indicate that successful maintenance of reduced body weight is associated with extremely high levels of dietary restraint [124, 125]. Whether this trait was present prior to the initiation of weight loss, or is a learned response, is the subject of ongoing research.
The Role of Leptin (See Fig. 7.1)
A critical mediator of these reciprocal changes in energy intake and expenditure is the adipocyte-derived hormone leptin that circulates in weight-stable individuals in close direct proportion to fat mass [126]. Leptin-deficient humans and rodents demonstrate a hyperphagic/hypometabolic phenotype that is similar to that seen in weight-reduced humans, and leptin signaling affects many of the weight-reduced phenotypes discussed above [127]. Leptin suppresses food intake by promoting the production of anorexigenic hypothalamic neuropeptides (processed products of POMC) and reducing the expression of orexigens such as neuropeptide Y (NPY), agouti-related peptide (AgRP), and melanin concentrating hormone (MCH). Mice overexpressing the melanocortin 4 receptor (MC4R) antagonists, agouti signaling protein (ASP) or agouti-related peptide (AgRP) [128] are obese. Thus, decreased circulating leptin concentration as a result of reduced fat and/or negative energy balance mass has the net effect of stimulating food intake [88].
The hypothalamic POMC–melanocortin–MC4R pathway is highly sensitive to circulating leptin concentrations and POMC expression is decreased in low-leptin states [129, 130] (see above). Therefore, reduced ambient leptin induced by weight loss should be associated with decreased HPT and increased HPA axis activity by virtue of decreased levels of hypothalamic α-MSH and β-EP, respectively [129, 130]. Rodents and humans with hypomorphic mutations in MC4R [131], disruptions of POMC gene expression [132, 133] or of proneuropeptide (e.g., POMC, pro-ACTH, pro-TRH) processing by prohormone convertases [134, 135] are obese. The importance of leptin in mediating these effects is confirmed in the observation that fasting in rodents causes hypoleptinemia that is associated with increased arcuate and brainstem NPY and AgRP mRNA expression and decreased POMC mRNA in lean animals to a greater degree than in leptin-receptor-deficient animals [136, 137]. Once activated, γ-aminobutyric acid (GABA-ergic) outflow from NPY neurons suppresses leptin activation of POMC and anorexiant neurons, including melanocortin-4 receptors. Leptin is also clearly not the only peripheral signals to NPY and POMC neurons. Caloric restriction increases NPY expression and decreases POMC expression in the arcuate nucleus as well as increasing corticosterone production in the obese, leptin-resistant Zucker fa/fa rat [138].
The effects of exogenous leptin on energy homeostasis are dependent upon the nutritional environment in which it is applied. Administration of leptin to leptin-deficient rodents and humans in doses that restore circulating leptin concentrations to their physiological range increases energy expenditure [139], decreases energy intake, increases sympathetic nervous system activity [140], and normalizes hypothalamic–pituitary–adrenal, thyroid, and gonadal function [88, 129, 141]. Yet, in humans (lean or obese) and rodents who are not leptin-deficient, induction of even modest weight loss requires doses of leptin that produce plasma leptin concentrations over ten times normal [142, 143]. In contrast to the limited effects on energy homeostasis of leptin administration to humans who are either leptin-sufficient or in a state of dynamic weight loss, leptin appears to have very potent effects on the hypometabolic, hyperphagic state that characterizes the weight-reduced and weight stable individual [54, 56, 141]. More specifically, we have found in short-term (5-week) studies that physiological leptin repletion following weight loss at least partially reverses the metabolic (decreased energy expenditure and increased skeletal muscle chemomechanical efficiency), neuroendocrine (decreased circulating concentrations of T3 and T4 but not TSH), and autonomic (decreased SNS but not increased PNS tone) [56]. In this sense, the weight-reduced state may be “perceived” by CNS elements relevant to energy homeostasis as a state of relative leptin deficiency. Pharmacotherapy designed to activate the leptin-signaling pathways may help weight-reduced individuals to sustain their weight loss [144]. It is likely that these agents will be effective at doses below those required for weight reduction per se.
Implications for Future Directions
Wing and Hill proposed that successful weight loss maintainers be defined as “individuals who have intentionally lost at least 10 % of their body weight and kept it off at least one year” [39]. No matter whether surgical, pharmacological, variations in diet caloric density or macronutrient content, or other behavioral methods are used to promote weight loss, it is apparent that for most individuals all or almost all of the weight loss occurs in the first 6–9 months of the intervention [145, 146]. While the amount of weight lost in this time period may vary (greatest with bypass bariatric surgery), the slope of the line relating subsequent weight regain to time after this initial weight loss period does not differ significantly among interventions [145, 147–149]. Approximately 15 years ago, McGuire et al. reported that only about 20 % of individuals who had attempted to lose weight were able to meet this goal of 10 % sustained weight loss [5].
These figures have not improved over the past decade and a half, despite the advent of multiple new weight loss and maintenance plans that are “guaranteed,” and the approval, and often subsequent disapproval, of various weight loss medications [150–152]. Kraschnewski et al. [6] reported that only one out of six overweight or obese adults is able to sustain a weight loss of 10 % or greater. The Look AHEAD trial examined the efficacy of an intensive lifestyle intervention in overweight or obese adults with type 2 diabetes that consisted of an approximately 1,600 kcal/day diet, 175 min of moderately vigorous physical activity per week, and screening by a health professional weekly for 6 months, then three times per month for 6 months, and then monthly for a total of 4 years. Only 40 % of the initial subject population was able to lose >10 % of their initial weight and of those, only 40 % were able to sustain the weight loss. Thus, even with an intense intervention, the odds ratio was 3:2 against being able to lose at least 10 % body weight and 6:1 against being able to lose and maintain a 10 % or greater reduced weight. The resistance to efficacy from this extensive and expensive intervention reflects, in part, the potency of the biological opposition to sustained weight loss.
These observations suggest possible directions for future research. Further research into means of extending the period of actual weight loss would result in greater initial reduction in body weight and a higher percentage of individuals reaching a weight at which co-morbidities, or co-morbidity risks, are significantly reduced. Dynamic weight loss, which is a state of negative energy balance, should be seen as a state distinct from static weight maintenance which is a state of energy balance. Pharmacotherapies designed specifically for weight maintenance, probably affecting the leptin signaling pathway, should result in reversal of much of the weight-reduced phenotype and assist in maintenance of reduced body weight. Other therapies, whether diet, exercise, pharmacologically, or surgically based should be specifically designed to reverse the consequences of weight loss with a particular focus on decreasing skeletal muscle work efficiency and appetite. Interventions, such as leptin, that are less effective in promoting weight loss in humans may be very effective in promoting maintenance of reduced body weight. The metabolic and behavioral responses to weight maintenance are both heritable and heterogeneous [19, 31–33, 153]. It may be possible to identify certain behavioral and imaging phenotypes and genotypes as well as environmental factors that are predictive of the weight loss and maintenance of reduced weight in response to different types of interventions.
There is substantial evidence that these questions can be answered. The pharmaceutical industry is examining combination medications to address concerns regarding weight maintenance and prolonging of the weight loss period [144]. Analysis of feeding behavior in gastric bypass patients indicates a significant post-operative decline in food reward value compared to gastric banding patients which could reflect changes in molecular signaling by intestinal peptides, changes in the gut microbiome, or learned behaviors following this surgery [154]. Cluster analyses of individuals successful at keeping weight off has suggested that the age of onset of obesity may be negatively correlated with likelihood of successfully losing weight and sustaining weight loss by behavioral intervention [42
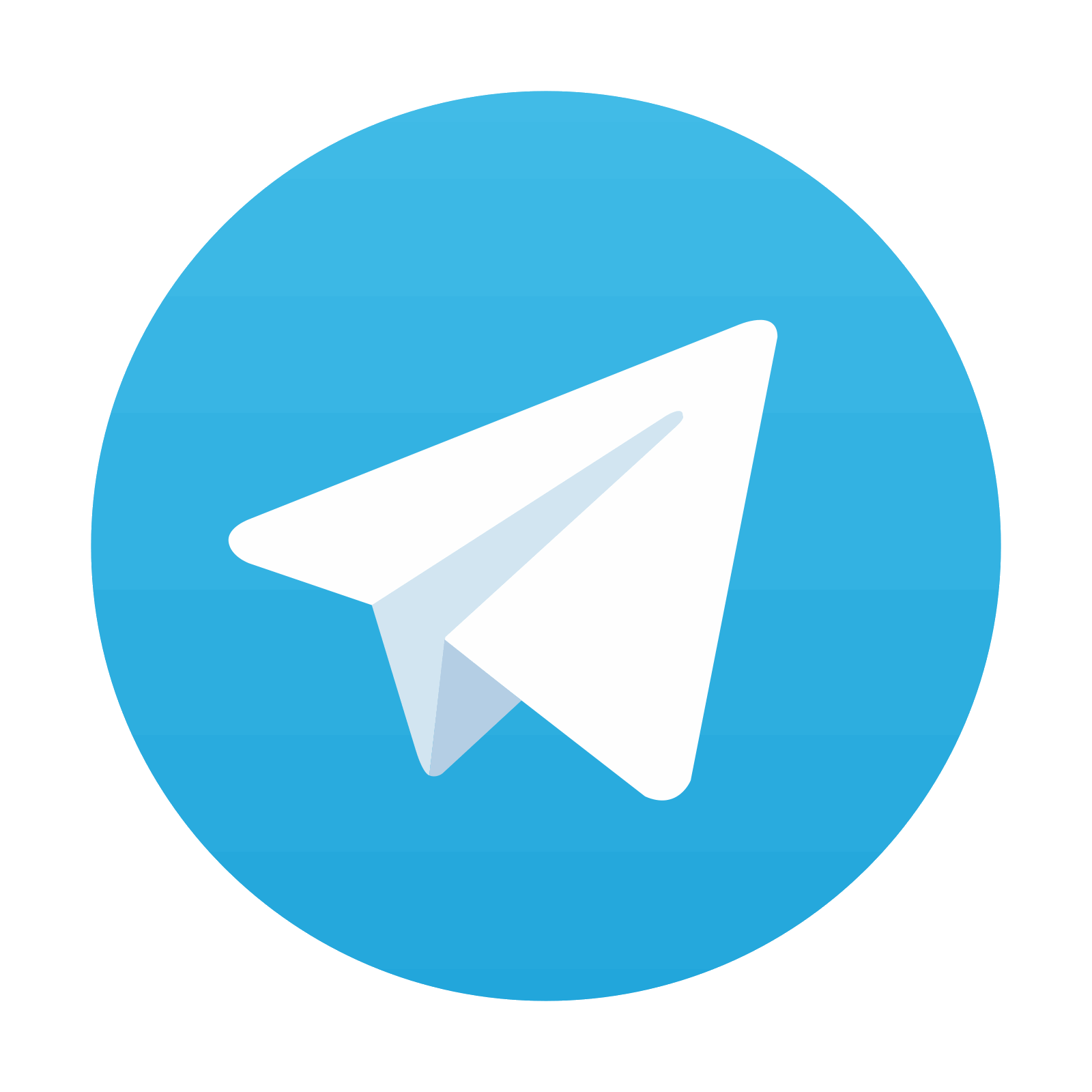
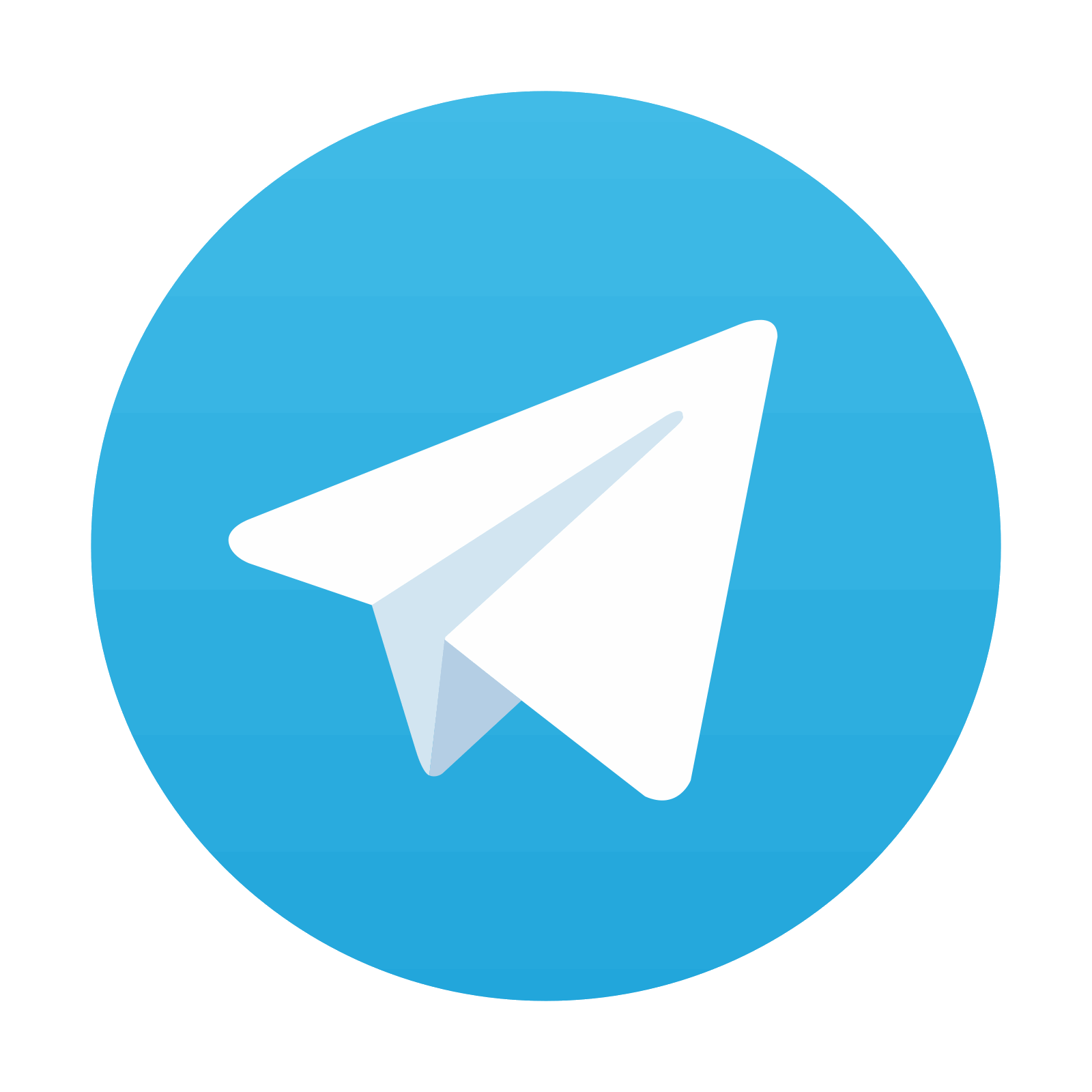
Stay updated, free articles. Join our Telegram channel
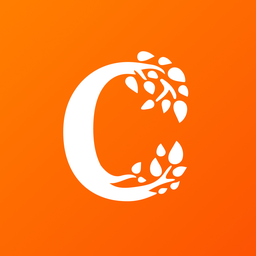
Full access? Get Clinical Tree
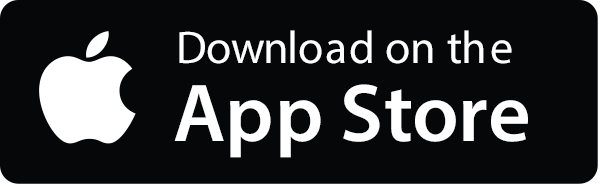
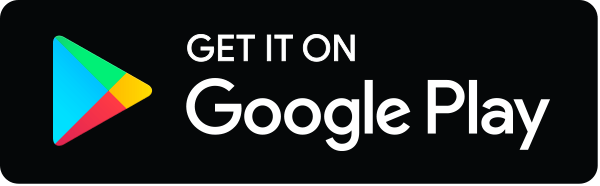